Quanta image sensor technology boosts emerging detector imaging capabilities via high-resolution, high-speed, and low-power linear photon counting at room temperature.
Dakota Robledo, GIGAJOT TECHNOLOGY
While CMOS imagers
have evolved significantly since the 1960s, photon-counting sensitivity has still required the use of specialized sensors that often come with detrimental drawbacks. This changed recently with the emergence of new quanta image sensor (QIS) technology, which pushes CMOS imaging capabilities to their fundamental limit while also delivering high-resolution, high-speed, and low-power linear photon counting at room temperature.
First proposed in 2005 by Eric Fossum, who pioneered the CMOS imaging sensor, the QIS paradigm envisioned a large array of specialized pixels, called jots, that are able to accurately detect single photons at a very fast frame rate1. The technology’s unique combination of high resolution, high sensitivity, and high frame rate enables imaging capabilities that were previously impossible to achieve.
The concept was also expanded further to include multibit QIS, wherein the jots can reliably enumerate more than a single photon. As a result, quanta image sensors can be used in higher light scenarios, versus other single-photon detectors, without saturating the pixels. The multibit QIS concept has already resulted in new sensor architectures using photon number resolution, with sufficient photon capacity for high-dynamic-range imaging, and the ability to achieve competitive frame rates.
Photon counting and number resolving
Image sensors capable of extremely low-light imaging or photon counting are sought after for applications in areas including the life sciences, medical diagnostics, astronomy, and quantum technology. While the capability to resolve the number of photons would be ideal for these applications, such sensors have not been commercially available. Furthermore, the detectors used in these applications must strike a fine balance
between sensitivity, spatial resolution, and temporal resolution to achieve optimal performance.
Commonly used photon-counting detectors include photomultiplier tubes (PMTs), single-photon avalanche diodes (SPADs), and electron multiplying CCDs (EMCCDs). These architectures employ impact ionization to generate a significant amount of photo charge when a single photon is absorbed, which makes the resulting amplified signal easy to detect over the detector’s background electronic noise.
While impact ionization greatly improves the sensitivity of a single pixel, implementing this technique generally requires increased pixel size, operating voltage, and system complexity, as well as reductions in spatial resolution, pixel fill factor, and quantum efficiency.
A smaller pixel size will yield a higher spatial resolution, but generally at the cost of a lower light signal and signal-to-noise ratio per frame per pixel in conventional cameras.
In contrast, the QIS technology recently developed by Gigajot Technology does not utilize impact ionization (Figure 1). Instead, it uses a patented pixel-device design to reduce the capacitance associated with the pixel sense node and passively increase the signal per collected photo charge. This technique can be implemented in small pixels measuring less than 1 µm and can support high-speed readout. This QIS technology has produced experimentally verified photon-counting pixels with sufficient sensitivity
to reliably detect and resolve every photo charge, overcoming the traditional trade-off between spatial resolution and low-light imaging performance (Figure 2). Importantly, quanta image sensors can also be manufactured using standard
commercial CMOS image sensor processes.
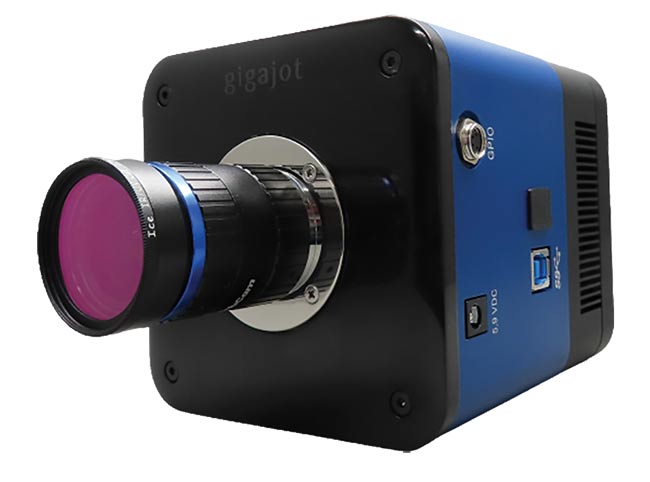
Figure 1. A quanta image sensor (QIS)-based camera capable of photon number resolving at full speed with a high spatial resolution (top). A QIS sensor chip designed and fabricated in a commercial CMOS image sensor process (bottom). Courtesy of Gigajot Technology.
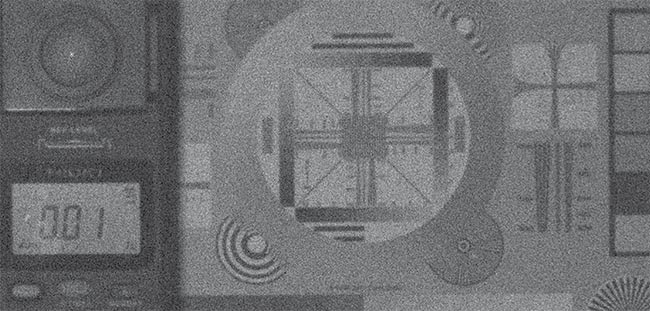
Figure 2. A low-light imaging comparison of an image captured using Gigajot’s GJ01611 QIS detector with 16.7-MP resolution and 1.1-µm pixel size (top) and an image captured using a state-of-the-art CMOS image sensor with 6.4-MP resolution and 2.4-µm pixel size (bottom). Images were captured under 10-mLux illumination with 40-msec exposure time through an f/1.4 lens. Both images show raw data without image enhancement. Though the QIS detector’s pixels are 4.8× smaller, its photon-counting read noise still delivers improved low-light imaging performance compared to the CMOS sensor. Courtesy of Gigajot Technology.
Read noise and pixel response
Read noise is a general term used to
describe the temporal noise introduced
by an image sensor’s readout circuitry.
It mainly consists of thermal, flicker,
random telegraph, and pixel reset noise. The reset noise, however, is typically
removed from the data by using correlated double sampling2.
Thermal noise is found in all forms of electronics due to the random fluctuations of charge carriers that result in small fluctuations in current over time3. Flicker noise is also inherent to all electronics, and it occurs in other parts of nature; its root cause is still widely debated4. Random telegraph noise is only found in a small percentage (0.001%) of pixels in an image sensor, and it is caused by imperfections during the fabrication process. In addition to maximizing the voltage signal per collected photo charge, care must be taken to minimize the impact of these noise sources.
Additionally, photon emission as described by Poisson statistics underscores that the number of photons received by a detector will not be uniformly distributed over time or space. Thus, even under
stable and flat illumination, each pixel in a frame will potentially measure a different integer value of photons. This fluctuation is often called photon shot noise.
Figure 3a shows the ideal response from a detector without any read noise for an average illumination of two photo charges per frame. For an image sensor with read noise, σn, the Poisson-distributed output signal will be corrupted by the Gaussian-distributed read noise. Figure 3b shows the same Poisson distribution as Figure 3a after the signal has been corrupted by various levels of read noise.
Photon-counting requirements
Figure 3b makes it quite clear that read noise is a key parameter in describing the performance of a photon-counting detector. As read noise decreases, the resulting distribution becomes more reflective of the underlying Poisson distribution5. In Figure 3a, recovering the number of photons received per pixel is quite simple because the output signal levels are discretely tied to an integer number of photons. The results shown in Figure 3b, however, indicate that if read noise is not zero, then performing this same quantization on a real device would lead to errors in the total photon number count.

Figure 3. The output signal distribution for an ideal image sensor with no read noise (top), and a realistic image sensor with various read noise values for an average light intensity of two photo charges per frame (bottom). Courtesy of Gigajot Technology.
The photon-counting error rate of a detector is often quantified by the bit error rate. The broadening of signals associated with various photo charge numbers causes the peaks and valleys in the overall distribution to become less distinct, and eventually to be indistinguishable. The bit error rate measures the fraction of false positive and false negative photon counts compared to the total photon count in each signal bin. Figure 4 shows the predicted bit error rate of a detector as a function of the read noise, which demonstrates the rapid rate reduction that occurs for very low-noise sensors.
Figure 4. Predicted photon-counting error rates for an average light intensity of one photo charge per measurement, with annotated error rates for 0.2, 0.5, and 1.0 e¯ RMS read noise. Courtesy of Gigajot Technology.
In addition to read noise, dark current can also affect the photon-counting accuracy of a detector. These silicon detectors are unable to determine the difference between a charge that is thermally generated and one that is photon generated. Therefore, if the dark current per frame is not significantly less than the light intensity, substantial data processing will be required to recover the actual incident light level.
Furthermore, dark current generation
follows Poisson statistics similar to the way photon emission does. Thus, dark current shot noise will effectively increase the read noise of the detector and can result in the loss of photon-counting accuracy. To avoid this, conventional scientific camera modules often employ a complex cooling system to ensure that the dark current remains at reasonable levels. Even with the added cooling, some scientific camera modules still exhibit amplifier glow, which typically has a strong spatial dependence and also limits the photon-counting accuracy. Gigajot’s quanta image sensors, in addition to the sensors’ low dark current, do not show any signs of amplifier glow.
Quantum efficiency is another key parameter that helps to define the real-world usability of a detector. Its optimization relies, in part, on how well the layout of the pixel can be designed so that photo charges are not lost before they can be collected by individual pixels. This is a common problem in SPAD-based detectors, as a portion of photons are often absorbed without triggering an avalanche event. If the detector has a low read noise as well as low effective quantum efficiency, then the signal-to-noise ratio will not be sufficient for low-light scenarios.
QIS vs. the state of the art
QIS-based detectors with 16.7- and
4.2-MP resolutions recently emerged on the market. They introduced new low-light imaging capabilities, with interesting implications for scientific imaging6-8 (Figure 5). Both detectors enable linear photon-counting at room temperature, along with full-speed readout, high spatial resolution, and a high dynamic range — all in a small form factor. This combination of industry-leading performance specifications makes the QIS
stand out from the other available photon-counting technologies such as SPADs and EMCCDs.
Figure 5. A sample fluorescence image of normal human colon epithelial cells tagged with the BioTracker 488 Green Nuclear Dye and captured with a QIS detector. The signal distribution
of the entire 16.7-MP array shows a clearly quantized photon-counting histogram with a strong peak-to-valley separation. DN: digital number. Courtesy of Gigajot Technology.
When discussing QIS technology in the context of other single-photon detectors, it is also important to distinguish between photon-counting sensors and those with single-photon sensitivity. Electron-multiplication-based detectors, such as EMCCDs or SPADs, can detect a single photo charge, but they cannot accurately resolve the number of photo charges received in each detection cycle.
The pixels in SPAD devices also need to be re-biased after each avalanche event, which leads to pixel dead time. Also, the output signal of a SPAD saturates when multiple photons are received in quick succession, which can increase the photon-counting errors.
High-Level Summary of Various Photon-Counting Detector Architectures
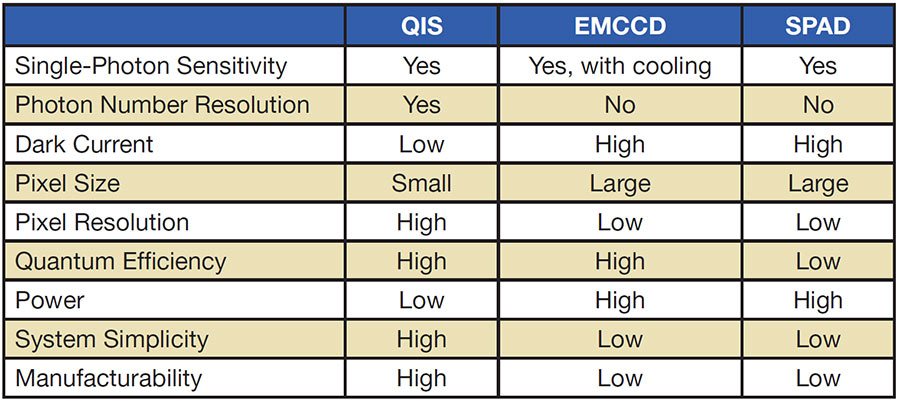
In an EMCCD, avalanche multiplication is applied during the charge readout process, usually by sending the charge through hundreds of multiplication stages. This process can cause the pixels to suffer from the excess noise that is induced by the stochastic multiplication gain, and it means that there is no accurate method for determining — based on the output signal level — how many photons are received by each pixel.
The table on this page summarizes the performance of various photon-counting detector architectures. The ability of
QIS detectors to achieve photon number resolution sets them apart from avalanche-based detectors, which are fundamentally limited in their photon number-resolving accuracy, and their
accuracy is further compromised by higher dark current levels.
Gigajot’s QIS detectors, in comparison,
demonstrate a mean dark current as low as 0.0069 e¯/µm2/s at 20 °C, with the median dark current at 0 e¯/µm2/s. In other words, the majority of pixels have a dark current lower than the detectable level. This helps to explain how Gigajot’s quanta image sensors are able to achieve photon-counting sensitivity with long exposure times at room temperature, without external cooling.
Notably, a recently announced quantitative CMOS detector has also claimed to achieve photon number resolution9. While the detector is able to achieve an impressive read noise of 0.27 e¯ RMS, it requires external cooling to −20 °C and a frame rate reduction to 5 fps — a fraction of the full frame rate — to achieve this noise level. Again, QIS-based detectors are able to achieve photon-counting resolution at room temperature without any reduction in frame rate.
Meet the author
Dakota Robledo, Ph.D., is a senior image sensor scientist at Gigajot Technology. He is experienced in various aspects of CMOS image sensor design and implementation, from realizing application-specific use cases to integrated circuit design and verification. He has a doctorate from the Thayer School of Engineering at Dartmouth, where he helped to test and develop QIS technology.
References
1. E.R. Fossum et al. (2016). The quanta image sensor: every photon counts. Sensors,
Vol. 16, No. 8.
2. M.H. White et al. (1974). Characterization
of surface channel CCD image arrays at
low light levels. IEEE J Solid-State Circuits,
Vol. 9, No. 1, pp. 1-12.
3. A. van der Ziel (1968). Solid State Physical Electronics. Prentice Hall.
4. K.K. Hung et al. (March 1990). A unified model for the flicker noise in metal-oxide-semiconductor field-effect transistors.
IEEE Trans Electron Devices, Vol. 37,
No. 3, pp. 654-665.
5. E.R. Fossum (2016). Photon counting error rates in single-bit and multi-bit quanta
image sensors. IEEE J Electron Devices
Soc, Vol. 4, No. 3, pp. 136-143.
6. J. Ma et al. (June 2021). A 0.19e¯ rms read noise 16.7Mpixel stacked quanta image
sensor with 1.1 μm-pitch backside
illuminated pixels. IEEE Electron Device Lett, Vol. 42, No. 6, pp. 891-894, www.doi.org/10.1109/led.2021.3072842.
7. J. Ma et al. (2021). A photon-counting 4Mpixel stacked BSI quanta image sensor with 0.3e¯ read noise and 100dB single-exposure dynamic range. Presented at 2021 Symposium on VLSI Circuits, pp. 1-2, www.doi.org/10.23919/vlsicircuits52068.2021.9492410.
8. Gigajot Technology Inc. (2022), www.gigajot.tech/product.
9. Hamamatsu (2021). ORCA-Quest, pp. 1-9, www.hamamatsu.com/resources/pdf/sys/SCAS0151E_C15550-20UP.pdf.