Applications as disparate as multimodal imaging and deep-tissue studies benefit from higher peak power and other new performance benchmarks for one-box lasers.
David Armstrong, Coherent Inc.
Nonlinear microscopic imaging techniques
are playing key roles across many areas of life sciences research because they can
perform high-resolution, three-dimensional spatial imaging of specific chemical
targets (labeled and unlabeled) in real time and in living tissue. In turn, laser
manufacturers have simplified these techniques and broadened access to them by developing
“one box” ultrafast lasers that provide turnkey operation for microscopy.
At first, the emphasis of these lasers was to deliver high value
through ease of use and performance flexibility. But we are now seeing an increase
in laser diversity to offer the highest possible performance on several fronts
– for example, to support researchers who demand the very longest wavelength
possible, or the highest peak power or the highest power in the near-infrared (out
to 1.6 µm). Consequently, to select the best laser for a particular application,
it is vitally important to understand how performance is defined.
Laser parameters: wavelength, power, pulse width
The latest one-box Ti:sapphire (Ti:S) lasers provide a tuning
range as wide as 680 to 1080 nm, usually with push-button control to maximize their
flexibility (Figure 1). This enables the laser wavelength to be easily tuned to
the needs of quite different experiments, or to multiple fluorophores used in the
same experiment.
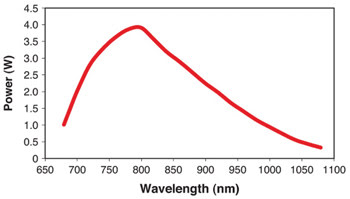
Figure 1. The wide tuning range of one-box Ti:sapphire lasers provides
maximum flexibility, particularly for multiple users. This is the typical output
tuning curve for Coherent’s Chameleon Ultra. Courtesy of Coherent Inc.
Power in microscopy lasers is usually expressed as time-averaged
output power. As shown in Figure 1, the gain of Ti:S varies with wavelength, with
peak output efficiency at roughly 800 nm. Currently, one-box Ti:S lasers optimized
for high power (e.g., the Chameleon Ultra) can deliver more than 3.5 W. Average
power is important because it enables images to be obtained in a shorter time, supporting
faster frame rates for dynamic imaging. High average power is critical also for
deeper imaging and for reaching the long-wavelength “tail” of the Ti:S
gain curve to 1080 nm.
The other key laser parameters are peak power and pulse width.
In practice, the shortest pulse width is obtained by using a cavity designed for
short pulse width and cavity optics that precisely compensate for group velocity
dispersion (GVD). The bane of ultrafast lasers and their users, GVD is a quantitative
expression of the fact that, in any transparent material, there is dispersion; the
speed of light is wavelength-dependent. This dispersion must be compensated using
optics so that all the different wavelength components that make up the short pulse
have exactly the same round-trip time in the laser. Currently, one-box lasers optimized
for short-pulse performance (e.g., the Chameleon Vision S from Coherent) deliver
pulse widths as short as 70 fs.
Pulse width is important because it is a major factor in determining
the peak power. Specifically:
Peak power = Average power/(Pulse repetition rate
x Pulse width)
So, for a given laser power, shortening the pulse width increases
the peak power, and the efficiency of multiphoton excitation is a nonlinear function
of peak power.
In addition to considering these laser parameters, it is also
important to understand the role of a couple of useful accessories that can modify
performance in two key ways: GVD precompensation and a tunable optical parametric
oscillator (OPO).
GVD precompensation
Before it reaches the sample, the laser pulse has to pass through
several beam delivery optics as well as the microscope optics. All of these have
dispersion and create GVD, thereby broadening the pulse in time. This is particularly
problematic if the microscope contains one or more acousto-optic deflectors, which
tend to be highly dispersive.
There are two ways to address this issue – passive and active.
The simplest (and lowest cost) solution is to design the laser pulse width for an
optimum value to match the GVD of the most common microscopes. With very short pulses,
their high bandwidth means that they experience substantial broadening and can be
quite long at the sample, reducing their peak power. Conversely, very long pulses
experience less broadening because of their reduced spectral bandwidth. But these
pulses start off long and cannot be shortened beyond that initial pulse width. So
the trade-off between initial pulse width and minimizing acquired GVD means that
there is actually an optimum laser pulse width of 130 to 160 fs that produces minimal
pulse width at the sample for the most common multiphoton excitation microscopes.
A more rigorous approach is to use an optical accessory that imparts
negative GVD to the pulse leaving the laser, to counter the positive GVD of the
downstream optics. This is often integrated completely within the laser, as this
maximizes beam pointing stability. Also, for maximum flexibility, it’s better
to use a precompensator with a wide dynamic range that can be smoothly adjusted
from zero to a value high enough to compensate for the most dispersive microscope
– for example, one with twin acousto-optic deflectors. In addition, this enables
wide pulse duration flexibility for optimized performance in quite different experiments.
Reaching longer wavelengths with an OPO
An OPO can shift the Ti:S laser output to a longer wavelength,
providing continuously tunable coverage over the 1- to 1.6-µm spectral region for
deep-tissue imaging, for example. The OPO’s automated frequency doubling and
tripling options also enable this accessory to provide tunable visible pulses, including
complete coverage over the Ti:S gap from 540 to 680 nm.
In addition, the latest generation of OPOs uses a fan-poled nonlinear
crystal. Unlike OPOs based on earlier bulk crystals, a fan-poled crystal can be
pumped with a broad range of wavelengths. This means the output of the Ti:S laser
and the OPO can be independently tuned (Figure 2). This innovation enables simultaneous
excitation of two very different fluorophores as well as multimodal imaging studies,
using all the different types of nonlinear imaging techniques, including coherent
anti-Stokes Raman scattering (CARS), second- and third-harmonic generation imaging,
and multiphoton excitation.
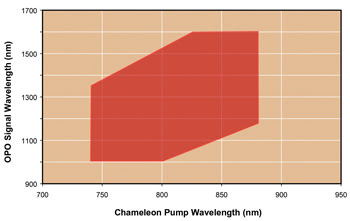
Figure 2. Fan-poled crystal technology provides independent tuning
as summarized in this graph, which shows the combination of simultaneous tuning
ranges provided by a Ti:sapphire laser and a fan-poled optical parametric oscillator.
(Chameleon™ Vision and Chameleon Compact OPO). Courtesy of Coherent Inc.
Which laser?
All commercial one-box lasers feature a reasonable tuning range
and beam quality, so choosing a laser model comes down to setting priorities for
the parameters where cutting-edge performance is most important.
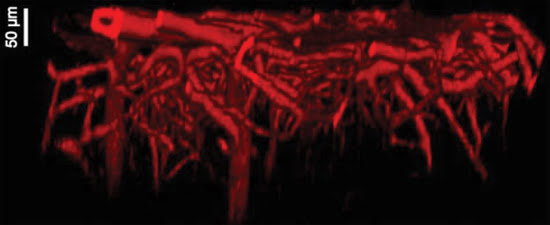
Figure 3. Long-wavelength excitation is critical for deep-tissue imaging, as illustrated
by this image of mouse brain capillaries extending to a depth of 250 μm. The
image was recorded with 1080-nm excitation using an Olympus objective and Coherent
Chameleon Vision. Courtesy of Dr. Hiroyuki Kawano, Professor Atsushi Miyawaki Lab,
Laboratory for Cell Function Dynamics, Brain Science Institute, Riken Institute,
Japan.
• Longest wavelength and GVD precompensation
The primary reason for prioritizing long wavelength is for deep
live-tissue imaging. Whether it’s to study some aspect of morphogenesis, to
model ischemic strokes or to study the murine cortex, live-tissue imaging is characterized
by the following: the need to minimize photodamage, the drive for deeper and faster
tissue imaging, and the need for higher signal-to-noise ratios. While the debate
about optimum pulse width and peak power continues, virtually everyone agrees that
all of these goals can be better met by ultrafast laser sources that deliver longer
wavelengths, close to or beyond the limit of Ti:S lasers, and that produce relatively
high powers at these long wavelengths. The use of longer (up to 1080 nm) wavelengths
results in reduced scatter losses and minimized photodamage, enabling deeper imaging.
Specifically, because of the homogeneous nature of biological samples, the majority
of light scatter scales nonlinearly with wavelength (specifically, 1/λn), so even
modest increases (by tens or hundreds of nanometers) in the excitation wavelength
can lead to significantly less scattering, which has proved to be a limiting factor
in deep-tissue imaging. This depth is further extended with GVD precompensation
(Figure 3).
The advantage of being able to optimize the pulse width at the
sample by GVD precompensation is illustrated in Figure 4, which shows two images
(with and without optimized laser precompensation) at a depth of 140 µm in a pituitary
gland sample labeled with green fluorescent protein and excited at a wavelength
of 930 nm.
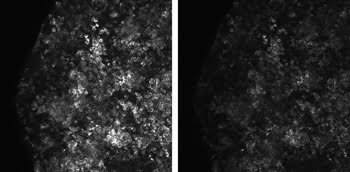
Figure 4. Two images of a pituitary gland
sample labeled with green fluorescent protein show the effects of optimized group
velocity dispersion precompensation (left) versus no precompensation (right). Images
recorded with a Zeiss LSM 7 MP microscope and a Coherent Chameleon Vision laser.
Courtesy of Jean-Michel Lago, Carl Zeiss sas.
• Highest power
The main reason for emphasizing high power is to increase the
efficiency of nonlinear processes, particularly the OPO at longer wavelengths, i.e.,
closer to 1.6 µm. The primary drivers are the widest wavelength flexibility for
multiple users as well as groups performing multimodal imaging, including CARS,
second- and third-harmonic generation imaging, and multiphoton excitation. These
techniques are all optimal for imaging different types of structures and molecules.
For example, CARS is often the best method for imaging lipids with a typical spectroscopic
interval of 2840 cm–1 to detect C-H stretching vibrations. Multiphoton excitation,
on the other hand, is ideal for exciting fluorophores as well as for endogenous
fluorescence to map the fate of genes and their protein products. And second- and
third-harmonic generation imaging methods are well-suited for imaging membranes
as well as collagen and muscle fibers and also can be used for some lipids. A typical
user in this category is professor Ji-Xin Cheng’s group at Purdue University
in West Lafayette, Ind., which uses multimodal imaging, including CARS, “to
enable label-free biological imaging based on the intrinsic signals from molecular
absorption and bond vibration,” according to the university website describing
his work (Figure 5).
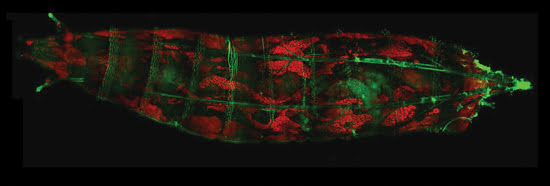
Figure 5. Multimodal coherent anti-Stokes Raman scattering/two-photon
fluorescence image, recorded with Coherent Chameleon Ultra and OPO, of Drosophila
larval fat body. Courtesy of Mikhail Slipchenko (Ji-Xin Cheng lab) and Wei Dou (David
Umulis lab) of Purdue University.
• Shortest pulses
In microscopy applications, shorter pulses deliver higher peak
power for brighter images from second- and third-harmonic generation imaging as
well as multiphoton excitation. The impact of shorter pulses can be understood by
comparing two different Coherent lasers, both featuring precompensation. The first
is optimized for shorter pulse width and delivers an average power of more than
2.3 W, while the second laser, optimized for other parameters, can produce up to
3.0 W. While this is a difference of only 20 percent in average power, the pulse
width of the first laser is 70 versus 140 fs for the second laser, resulting in
a 50 percent increase in peak power.
Who should prioritize short pulse width? This is a somewhat contentious
issue. With fixed tissue, the answer is simple: anyone who is dealing with potentially
very weak images – for example, deeper images based on third-harmonic generation.
With living tissue, there are two schools of thought. Some researchers prefer very
short pulse widths because this undoubtedly increases signal strength, which scales
as peak power2 or even peak power3, for three-photon effects (multiphoton excitation,
third-harmonic generation). But others claim that since tissue damage also scales
as a high order of the laser peak power, shorter pulse widths are not an advantage,
and they believe that adjustable average power is a more important feature.
The success and growth of nonlinear imaging techniques have been
enabled by turnkey lasers that offer users maximum flexibility. But, as researchers
refine these techniques, continued progress will require lasers that push the limits
of performance, without sacrificing reliability or ease of use.
Meet the author
David Armstrong is product line manager at Coherent Inc. in Glasgow,
UK; e-mail: [email protected].