Software control of system dispersion provides pulse stability and unleashes the potential for industrial applications of ultrafast lasers.
Adam Tanous and Dr. Mike Mielke, Raydiance Inc.
The minute details of high-energy ultrafast pulse production are complex, but the process comprises four basic steps. In the first step, a seed laser – generally a mode-locked laser – generates relatively low energy pulses of 500 fs. Dispersion is the second step: The pulses are stretched out to give them longer duration (about 1 ns), making them broader but giving them lower peak power. In the third step, the pulses are amplified to higher energies and, in the final step, the broad but now higher-energy pulses are compressed in time, which is essentially a reversal of the earlier dispersion.
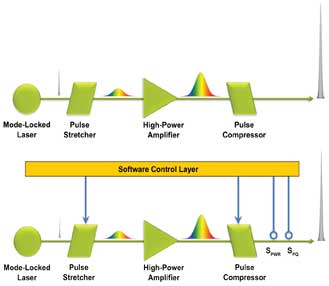
Figure 1 (Top). Schematic illustration of the chirped pulse amplification process. Low-energy pulses are generated by a seed laser. They are then stretched in time, amplified and compressed down to 700-fs pulses with 50 μJ per pulse. Images courtesy of Raydiance Inc. Figure 2 (Bottom). Chirped pulse amplification with feedback loop that monitors pulse quality and adjusts components in real time to manage pulse width stability. SPWR and SPQ refer to the power and pulse quality sensors, respectively.
Chirped pulse amplification, the result of this process, is a stream of pulses with both short duration and high energy (Figure 1). This intensity enables athermal ablation of materials such as bioabsorbable polymers or noble metals, both of which are used in medical device applications and are difficult to machine.
This technique is well understood but, in practice, the two manipulations of the pulses – stretching and then compressing them – have to be perfectly balanced. And even slight changes in ambient temperature or operating conditions can disturb the balance, producing pulses that are too broad for optimal athermal machining. For traditional ultrafast lasers, a change in temperature of even 1 or 2 °C can broaden the pulse by a factor of two or more. A laser originally operating in an athermal regime (subpicosecond pulse width) would, rather, be operating at 2 or 3 ps and introducing heat to the target. Such unpredictability in pulse width will result in unpredictable machining precision and manufacturing quality.
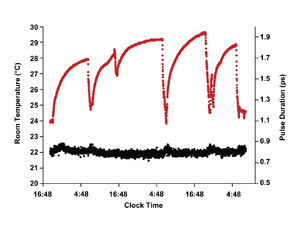
Figure 3. Pulse width (FWHM) stability measured over 60 hours and with normal ambient temperature fluctuations. The relative pulse width fluctuation was 5.7 percent.
Traditionally, this pulse width variability has been addressed either by maintaining very tight control of operating environments, or by assigning a laser engineer to constantly “tune” the system. Both approaches are costly.
Active pulse management
A new technology called active pulse management from Raydiance Inc. of Petaluma, Calif., integrates hardware and software components to enable stable pulse width in the face of ambient temperature changes and varied laser settings such as output power. Active pulse management technology comprises integrated actuators, sensors and embedded software control that maintain precise balance in dispersion, without the need for constant tuning (Figure 2).
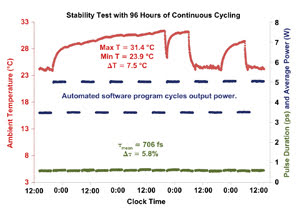
Figure 4. Pulse width stability (green) with temperature fluctuations (red) and programmed average power cycling (blue). Relative pulse width fluctuation over 96 hours was 5.8 percent.
In the system, power and pulse quality sensors monitor the laser output from the pulse compressor and provide simple electronic signals to be fed into the Raydiance operating system. Actuators integrated into the stretcher or compressor proportionally adjust the system dispersion in real time with no effect on the laser application process or laser output disruption.
Stability measurements
To evaluate the effectiveness of the pulse management system as well as the overall control and optimization system, Raydiance conducted a number of ISO standard stability tests (ISO 11554). In all cases, the relative fluctuation of a given variable was measured. For instance, the relative fluctuation of pulse energy, ΔQ, is defined by 2σ/Qmean, where σ is the standard deviation and Qmean is the mean pulse energy over the measurement period. Key parameters measured included pulse width stability with ambient temperature fluctuations and power cycling, pulse energy stability, average power stability with ambient temperature fluctuations, average power stability with continuous operation, and pulse quality over time.
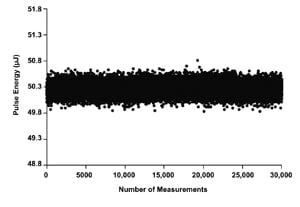
Figure 5. Pulse energy was measured 30,000 times consecutively. Relative fluctuation, ΔQ, was 0.43 percent.
Pulse width, energy stability
Pulse width (full width half maximum) was measured over the course of 60 hours of continuous operation and with changes in the ambient temperature between 24 and 30 °C. The system was run at 50 μJ per pulse and 100 kHz (5 W). As can be seen from Figure 3, the mean pulse width was 801 fs, with a relative fluctuation of 5.7 percent. With similar temperature changes, a traditional femtosecond laser would typically show a fourfold increase in pulse width, resulting in thermal effects and a reduction in ablation efficiency.
In addition to temperature changes, a Raydiance system was subjected to software-controlled cycling of average power output over time. Over the course of this 96-hour test, the pulse width showed a relative fluctuation of 5.8 percent (Figure 4).
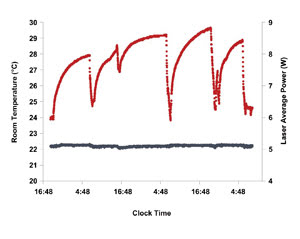
Figure 6. Average power output showed a fluctuation of 0.9 percent over a 60-hour test with 7 °C ambient temperature changes (red).
Running the Smart Light system at 50 μJ and 3 kHz, the pulse energy was measured 30,000 times consecutively. With mean pulse energy of 50.27 μJ, the relative fluctuation was 0.43 percent (Figure 5).
Average power stability
Average power output was measured over temperature changes similar to those earlier. Here again, the relative fluctuation of average power was very low: 0.9 percent over 60 hours of testing. The mean average power was 5.1 W (Figure 6).
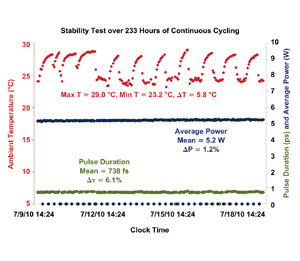
Figure 7. Average power output and pulse width measured over nine
days (233 hours) of continuous operation. The relative fluctuation of
the power was 1.2 percent; that of the pulse width was 6.1 percent.
The average power and pulse width were measured for more than nine days (233 hours) of continuous operation (Figure 7). The daily ambient temperature changes are shown in red. The laser was cycled off periodically (revealed as zero values in the power) to incorporate anticipated usage scenarios within a real workstation. The average power fluctuation over this period was 1.2 percent, and the pulse width fluctuation was 6.1 percent.
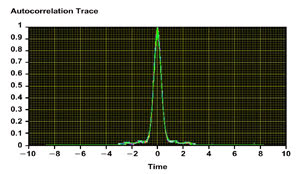
Figure 8. Autocorrelation traces taken over the course of 24 hours.
One hundred traces were taken and overlaid on one another. The
horizontal axis shows time measured in picoseconds. The pulses are
exceptionally consistent, despite a 7 °C ambient temperature
fluctuation.
Consistent high-quality pulse shape is critical to achieving consistent ablation precision. Energy that is delivered in pulses that vary in shape, or that are not well-confined in time, will introduce thermal effects and unpredictable ablation quality. To measure this parameter, 100 autocorrelation traces of the Smart Light pulse were taken over the course of 24 hours of operation. During this time, the temperature swing in the lab was 7 °C. As can be seen in Figure 8, the pulse traces overlap well.
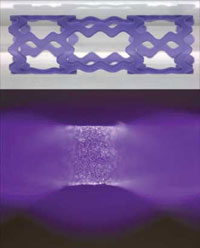
Figure 9. (top) A prototype PLLA stent with an outer diameter of
3.175 mm, machined with a Raydiance ultrafast laser employing active
pulse management. (bottom) A side view of a strut revealing good cut
quality and no heat-affected zone. The wall thickness is 254 μm.
Predictable pulses for applications
Active pulse management technology, because it ensures that an ultrafast system’s output has uniform pulse width and quality at all times, results in reliable athermal ablation for high-precision, high-yield materials machining – for micron and submicron processing of noble metals, dielectrics, semiconductors and heat-sensitive bioabsorbable polymers. Examples of the reproducible high-precision machining possible in relatively uncontrolled industrial environments can be seen in Figures 9 through 11.
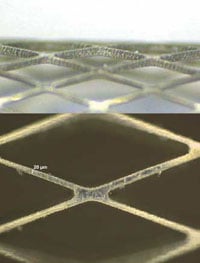
Figure 10. (top) A diamond pattern was machined in a 1.5-mm diameter nitinol tube. The wall thickness shown at left is 80 μm. (bottom) Individual struts are approximately 20 μm wide.
Ultrafast lasers, long used for dramatic, intriguing results in controlled laboratory venues, can be more widely deployed for commercial applications if it can be demonstrated that repeatable athermal results are possible even in conditions that are less than ideal. Real-time continuous software monitoring and adjustment of system dispersion will prove critical to realizing ultrafast lasers’ true commercial potential.
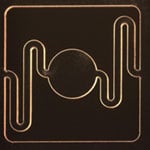
Figure 11. A microfluidic “check valve” machined in polycarbonate.
The central reservoir is approximately 1.5 mm in diameter. The
peripheral channels are approximately 234 μm wide.
Meet the authors
Adam Tanous is director of marketing at Raydiance Inc.; e-mail: [email protected]. Dr. Mike Mielke is chief scientist at Raydiance; e-mail: [email protected].