Dr. Nigel Gallaher, Darryl McCoy and Marco Arrigoni, Coherent Inc.
Genetically encoded probes are driving the development of customized lasers for this red-hot area of neuroscience.
The growing array of genetically encoded fluorescent probes is revolutionizing many areas of neuroscience, allowing researchers to move from topological to functional maps of the brain. In particular, optogenetics is enabling scientists to use light to activate or inhibit signaling among neurons and, in some cases, to link details of neural network operation to behavioral and sensory functions of the whole animal. This dynamic field has created a need for tailored ultrafast laser sources, such as fiber lasers, producing high power at wavelengths beyond 1000 nm.
Multiphoton imaging is a field that highlights excellent synergy between lasers and their applications. Starting from the first one-box Ti:sapphire lasers, new developments in the field, such as independent optical parametric oscillator (OPO) tuning and integrated pre-chirp, have all been introduced to better meet the specific needs of an evolving and dynamic branch of biophotonics. Optogenetics is yet another standout example of this trend, where existing technology can demonstrate a new technique that yields new science, but widespread deployment and better data can result only from new laser tools specifically developed and optimized for this application.
Optogenetics involves using light, often (but not always) in conjunction with microscopy, to activate or silence neural networks in the brain cortex of live animals that have been genetically modified to express these light-sensitive probes. In the most general case, an optogenetics experiment on the microscopic scale involves also imaging the neurons and probing the presence or absence of signaling activity (including timing) in the local neural network. The combination of these protocols is usually called all-optical physiology. Ultrafast lasers for multiphoton excitation (MPE) are ideal tools for all three protocols because MPE enables deep (1 to 1.5 mm) imaging with full 3-D resolution and low photodamage. This way, larger brain volumes can be studied in vivo for extended periods. The classic setup involves a mouse with a thin glass cover replacing a small part of the skull to provide visual access to the cortex at layers I through VI.
Stimulating neurons: Optogenetics began with the expression of light-sensitive proteins called opsins in specific types of neurons to enable light of an appropriate wavelength to modify the activity of these neurons. Specifically, because light stimulation of these proteins changes the cation (usually Ca2+) gradient, and hence the electrical gradient, across cellular membranes, targeted local illumination can stimulate or silence activity in the target neuron(s). This soon led to the adoption of channelrhodopsins (e.g., ChR1 and ChR2) and whole other classes of light-sensitive genetically encoded tools to control local ionic concentrations.
Probing neurons: The other key part of an optogenetics experiment performed on a microscopic scale is observing how the activation or silencing of a neuron affects the interconnected neurons. Again, multiphoton laser excitation provides the solution. Here, the calcium-ion concentration changes associated with neuron activity are imaged using reversible indicators whose fluorescent properties are directly related to the local concentration of Ca2+ or other relevant ions. The most common probes of this type, called genetically encoded calcium indicators (GECIs), are the GCaMP family; neurons expressing these probes show up as bright in the presence of Ca2+ and as dark in its absence. In the future, it may even be possible to genetically express probes that fluoresce in direct response to the actual voltage (called action potential) propagating in the neuron. These probes would have the advantage of a much faster response time than GECIs.
Imaging neurons
Stimulating and/or probing a set of neurons by laser excitation is usually preceded by imaging the cube of tissue that encompasses all neurons of possible interest. Green fluorescent protein (GFP) and its derivatives are the most common genetically encoded imaging probes, expanded in the past 10 years to include longer-wavelength probes such as the mFruit series. Standard MPE imaging is usually employed, raster scanning a section of the sample and stepping the plane of focus to build up a high-resolution 3-D image.
Avoiding crosstalk with tunable sources
The most advanced experimental in vivo sequence based on MPE involves first imaging the neural network to acquire and map the positions of the neurons. Then, one or more targeted neurons are stimulated while the same or other neurons are probed within the timescale required by the experiment and as limited by the speed of the microscope’s imaging technology. To obtain useful data, it is important to minimize crosstalk between the stimulation and probing phases of the experiment. This is achieved by using two laser wavelengths within the same microscope setup.
Figure 1 shows schematically how this works. Two ultrafast laser wavelengths are focused into the sample. Wavelength 1 is used to activate neurons by two-photon excitation, and Wavelength 2 is used to image activity in other neurons, again via two-photon excitation. The initial structure imaging is usually also done with two-photon and often using a fluorescent protein that works at the photoactivation wavelength, because activating many neurons simultaneously has no impact on the topological image. Moreover, this simplifies the experiment because it requires only two, rather than three, separate wavelengths.
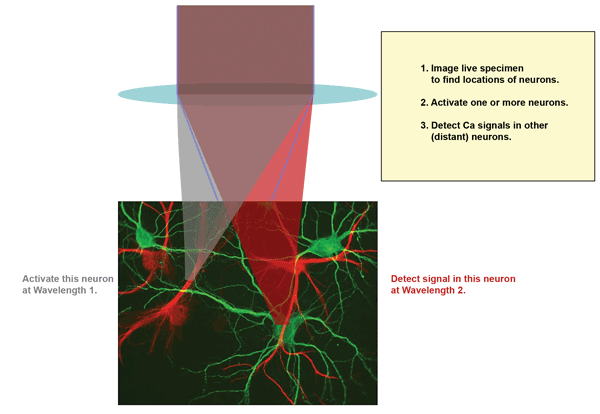
Figure 1. A common experimental scenario involves multiphoton excitation (MPE) imaging to map the neurons, followed by activation of one or more neurons using MPE at Wavelength 1, and probing of Ca2+ activity in remote neurons by MPE at Wavelength 2. Wavelength 1 (or sometimes Wavelength 2) is also used for the initial MPE mapping. Images courtesy of Coherent Inc.
The choice of wavelengths is determined by the need to avoid crosstalk, between activation/silencing and probing, as well as the spectral characteristics of the available control and probe tools (e.g., ChR1, GECIs), together with the outputs available from the laser source(s).
All-optical physiology experiments involving optogenetic methods have been performed using a one-box Ti:sapphire ultrafast laser (Coherent’s Chameleon) with an independently tunable OPO to generate the two necessary wavelengths. The use of a so-called fan-poled crystal in the Chameleon OPO enables a unique degree of independent tuning of the two wavelengths – from the laser oscillator and the OPO (For more details, see “Neuroscience Drives Advances in Lasers for Multiphoton Imaging” in the October 2013 issue of BioPhotonics).
What are the specific wavelengths needed? As shown in Figure 2, one approach is to use 950 nm as Wavelength 1. This is optimal for activating ChR2 and close enough to 920 nm to produce the initial MPE image based on GFP. However, some of the best activity probes (the GCaMP family) are also optimally excited by two-photon absorption at 950 nm. So when using ChR2, a red-shifted calcium indicator such as RCaMP must be used. But these red-shifted indicators are still in their infancy, and their optical properties are not yet fully optimized.
Alternative solutions include using dyes as Ca2+ indicators; Oregon Green, for example, can be excited at ~850 nm in conjunction with ChR2. Minimization of optical damage and optimization of imaging depth, however, recommend the use of longer wavelengths; furthermore, it is desirable to use all genetically encoded probes. Fortunately, some longer-wave-length optogenetics activators are well developed and stable, such as C1V1, which can be activated effectively at 1050 nm. Probing can then be done at 920 nm with GCaMP, which is unresponsive to 1050 nm. In Figure 2, this combination is shown as Example 2.
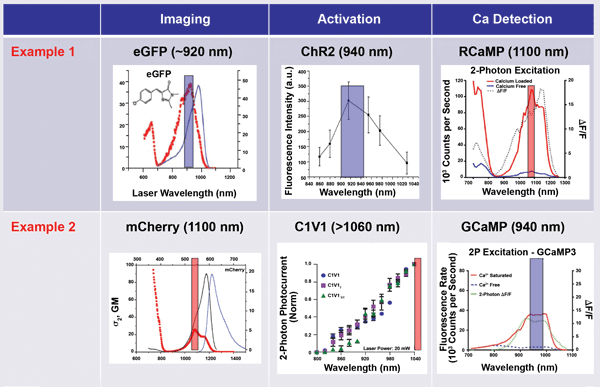
Figure 2. In optogenetic imaging, it is vital to avoid crosstalk between the activation (control) phase and the probe phase. This is done by wavelength separation. In Example 1, a short wavelength is used for the activation (and initial passive imaging), and a long wavelength is used for two-photon excitation of the probe. In Example 2, a long wavelength is used for imaging and activation, and a short wavelength probe monitors neuron activity. Adapted from various sources (The colored bar is ~50 nm wide, centered at the peak of the excitation spectrum). eGFP = enhanced green fluorescent protein; ΔF/F = relative change in fluorescence signal; σ2, GM = two-photon absorption cross section, σ2, measured in Goeppert-Mayer units; 2P = two-photon.
Existing commercial solutions using a Ti:sapphire or other pump laser, together with an OPO, can produce paired wavelength combinations that can excite both GCaMP indicators and red-shifted opsins. Where image intensity and/or speed are not critical requirements, this combination provides the simplest approach to all-optical physiology. However, the total available power from the combination of the OPO and its pump is about 1 W. Although this output power is perfectly adequate for conventional raster-scan MPE imaging, trends in advanced optogenetics and optical physiology require fast point-to-point imaging techniques or simultaneous multipoint approaches that are both power-hungry, for reasons described in the following section.
1050 nm: higher power, operational simplicity
Wavelengths beyond 1000 nm are advantageous for optogenetic activation of probes like C1V1, but these long wavelengths are near the end of the tuning range of Ti:sapphire lasers, where they can’t produce much power. And laser power is important for optogenetics, for several reasons. The emission of fluorescent probes (when not saturated) is proportional to the laser peak power times the average power. Brighter images with higher signal-to-noise ratios can enable deeper images, where the maximum depth is ultimately limited by scatter. Looking to the future, power is needed for faster speed. Specifically, at present, a typical multiphoton experiment with individual neuron resolution might involve stimulating and then imaging the activity of only a few neurons simultaneously. Ultimately, researchers would like to survey as many as 10,000 neurons at the same time – which means a column of cortex measuring 250 × 250 µm with a depth of up to 1 mm. This will require very fast scanning with fast modulators, or splitting the beam intensity and scanning the tissue with multiple focused spots. Either way, power will be key to obtaining acceptable signal-to-noise images at these faster sampling speeds.
Therefore, a clearly defined need exists in optogenetics for a dedicated source of higher power (greater than 1 W) at greater than 1000 nm, to be used as a stand-alone excitation source or in conjunction with a Ti:sapphire laser. Of course, with the high direct and indirect costs of today’s research, scientists want this power in a format that does not add undue cost or complexity, and does not sacrifice the short pulse width (brighter images) characteristic of Ti:sapphire sources.
New laser for optogenetics
In response, laser manufacturers have developed a new type of fixed-wavelength laser based on cost-effective and reliable ytterbium-doped fiber technology, and featuring operational simplicity and sealed-box performance. This fiber-based technology is optically simpler than Ti:sapphire lasers, and can be economically packaged in a compact and extremely rugged, zero-maintenance sealed laser head. In the past, ultrafast fiber lasers based on ytterbium or erbium (as well as bulk lasers based on ytterbium) faced a trade-off between power and pulse duration; it has been nearly impossible to produce a sub-100-fs laser with power in excess of a few hundred milliwatts.
Fortunately, this hurdle has now been overcome. An example of this next-generation ultrafast fiber laser is the new Fidelity from Coherent (Figure 3), which delivers over 2 W of power at 1055 nm in short (<70 fs) pulses. Moreover, the hands-free operation and long-term stability of this closed-box laser are an example of Coherent’s so-called industrial revolution in ultrafast lasers, where design and manufacturing practices from industrial lasers are being deployed into scientific lasers to deliver improved reliability, 24/7 performance and higher overall data productivity.
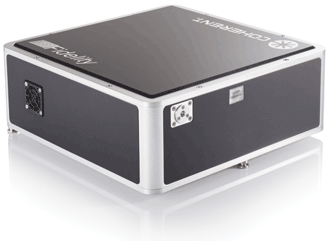
Figure 3. New compact, rugged ultrafast sources based on fiber technology, such as the Coherent Fidelity, are examples of the company’s latest design philosophy, dubbed “the industrial revolution in scientific lasers.”
Of course, a key goal for any live-tissue imaging tool is to deliver maximum image brightness with minimum total dosing – i.e., the brightest possible image for a given laser average power level. Image brightness is proportional to the product of peak power and average power. Higher peak power can be achieved either by increasing the pulse energy and/or decreasing the pulse width, as long as sample damage is avoided. The new laser meets this goal by delivering much shorter pulse widths (<70 fs) than other ultrafast fiber lasers (which are typically >200 fs) at a low-damage wavelength.
In addition, it is important to remember that the peak power and average power at the sample are what actually determine the brightness of MPE images. The broader spectral bandwidth of a 70-fs pulse means that group velocity dispersion in the beam delivery optics will stretch the initial laser pulse width. For this reason, the new laser incorporates a software-controlled precompensator to enable the user or system builder to offset downstream group velocity dispersion and therefore minimize the pulse width at the sample. Moreover, this feature allows researchers the option to smoothly vary the pulse width at the sample, which could be used to characterize trade-offs between photo-damage (such as photobleaching) and optimum imaging brightness.
The simultaneous application of Fidelity and a Chameleon to an all-optical physiology experiment at wavelengths of 900 to 920 nm and 1050 nm uniquely provides ~2 W of power at each wavelength, enabling fast, parallel imaging, activation (or silencing) and Ca2+ signal detection. In addition, this new fiber laser can also be used as a stand-alone excitation source for second-harmonic generation imaging, a technique that has shown potential for clinical applications to muscular and degenerative diseases, as well as cancer.
Meet the authors
Dr. Nigel Gallaher is a senior product manager at Coherent Inc. in Santa Clara, Calif.; email: [email protected]. Darryl McCoy is the director of product marketing at Coherent; email: [email protected]. Marco Arrigoni is marketing director for the scientific market at Coherent; email: [email protected].