Cicely Rathmell, Mapleseed LLC; Daniel D. Hickstein, Jila – University of Colorado Boulder; Craig Hanson, Idex Corporation
Laser-induced plasma generation in nanoparticles has potential application in fields as diverse as medicine and materials processing. To fully harness this tool, it is important to understand how nanoparticles behave in strong laser fields and how they can be controlled.
Science and engineering have always been about solving problems, and as those problems get bigger, the solution often is to make things smaller – sometimes much, much smaller. Nanomaterials behave differently from their bulk equivalents, as their size results in exceptionally high surface-to-volume ratio, inducing unusual quantum mechanical effects. Their novel properties are opening up a new dimension in materials design, with applications in energy production, medicine, communications, computing and more. Nanomaterials interact uniquely with light, as particularly strong electric field enhancements can occur when features are smaller than the wavelength of light. Consequently, new techniques are needed to observe the interaction of strong (damaging) laser fields with nanomaterials.
Recent experiments have shown that by observing nanoparticles flying through a vacuum chamber – rather than being suspended in liquid or bonded to a solid – it is possible to study the behavior of individual, isolated nanoparticles. When the particles are excited near the plasma threshold, the results are unexpected, revealing nanoscale field enhancements and the formation of localized nanoplasmas that could become the foundation of new techniques for observing and shaping materials on the nanoscale.
A golden combination
Most studies of the interaction between intense laser fields and nanomaterials have been performed on nanoparticles in solution or on a surface and are often subject to surface or solvent effects. In addition, these studies typically probe many nanoparticles at once, providing a picture of the average behavior of many nanoparticles. Variations in the size, shape, composition, surface structure and orientation of the nanoparticles are obscured, though these variations can have a significant impact on their response to light.
A new technique called plasma explosion imaging (PEI) has been developed at the University of Colorado Boulder to look at single nanoparticles irradiated by femtosecond pulses, exploring the diversity in their behaviors at intensities near the plasma threshold.1
Femtosecond lasers often are used to observe the dynamics of ultrafast processes or to stimulate nonlinear processes and generate plasmas. The timescale of a femtosecond pulse is faster than that of heat transfer, resulting in very local action, as with the clean features created in femtosecond machining of materials.
Applications combining ultrafast lasers and nanomaterials already are in development; for example, gold nanoparticles are being engineered to target cancerous tumors in the body for irradiation and selective destruction via shock wave formation using a femtosecond laser.2,3
Picking a single particle out of the crowd
Looking at single nanoparticles highlights the effect of variations in morphology and composition, offering clues to the mechanisms behind the behavior of nanoparticles in strong fields. But how, exactly, do you isolate a single nanoparticle to then observe it? It takes a combination of patience and probability. Nanoparticle aerosols are created from an aqueous solution using a compressed gas atomizer and are then introduced into a vacuum chamber as a collimated beam via an aerodynamic lens (Figure 1).
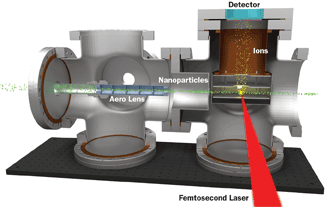
Figure 1. The plasma explosion imaging apparatus used to record photoion momentum distributions from nanoplasmas generated within nanoparticles. An aerodynamic lens focuses nanoparticles into a vacuum chamber, where they are irradiated by a femtosecond laser pulse. Ejected photoions are collected by a velocity map imaging spectrometer.
When irradiated with a tightly focused beam of 785-nm, 40-fs-pulse laser light, the limited interaction volume between the two beams results in irradiation of only one nanoparticle at a time, and only once every 100 or so laser pulses.
If the laser intensity is high enough to generate a plasma, ions are ejected, and their momenta can be recorded with a velocity map imaging (VMI) photoion spectrometer. This 2-D projection of the 3-D angular distributions of the ions yields information on both formation and location of the plasma within the nanoparticle.
When researchers first attempted the experiment, they were shocked at the strength of the signal. “Initially, we didn’t realize that we could form a plasma with these laser intensities, and we only expected to see a few ions ejected from each particle,” said Dr. Daniel Hickstein of the University of Colorado Boulder. “So we cranked the voltage on the detector way up while we attempted to align the laser to the particle beam. When we finally hit the first particle, it exploded into millions of ions: The detector lit up like a lightbulb, and all of the power supplies automatically shut down. We thought that we had just incinerated our $10,000 detector.”
When they turned down the voltage on the detector, they were excited to realize they were hitting individual nanoparticles.
Understanding the plasma threshold
Most dielectric materials have a damage threshold4 around 3 × 1013 W/cm2. Above this threshold, bulk materials irradiated with femtosecond pulses break down via an avalanche ionization process5-7 due to the strong electric field of the laser, similar to the way a strong electric field in the atmosphere ionizes air molecules to form a lightning bolt. Multiphoton or tunnel ionization generates free electrons that are accelerated by the strong laser field, in turn ionizing more atoms via electron impact ionization.
Both processes scale exponentially with laser intensity, and thus even a small increase in the field experienced can initiate plasma formation when close to the threshold.
Modeling the breakdown of nanomaterials in strong laser fields has been notoriously difficult, as optical properties of the material itself are affected by interaction with the laser field within femtoseconds. Finite-difference time-domain (FDTD) modeling has proved effective in predicting the behavior of nanoparticles irradiated below the damage threshold, particularly for subwavelength focusing and plasmonic effects. Exploring the regime near the plasma threshold can determine just how far FDTD models can be taken.
Nanoplasma formation
This is where things get interesting. Just as nanostructured substrates and colloidal solutions of gold or silver can enhance the Raman effect in surface-enhanced Raman scattering (SERS), the structural features of nanoparticles can enhance the electric field on a nanoscale level in many different ways. While the laser intensity applied may not be great enough to generate an avalanche ionization process throughout the nanoparticle, local enhancements of the electric field within the nanoparticle can exceed the damage threshold, forming a plasma with a temperature over 50,000 K (90,000 °F) or more in only those regions, causing electrons and ions to explode outward rapidly (Figure 2).
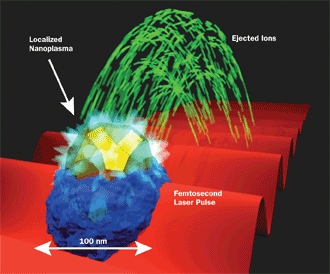
Figure 2. Nanoplasma formation in a nanoparticle caused by local enhancement of the electric field from a femtosecond laser (50-nm gold nanosphere embedded in a dielectric matrix).
At first it seems intuitive that the plasma would then propagate throughout the material. However the femtosecond laser pulse is so brief that even these fast-flying ions can propagate only a few nanometers before the laser pulse is over, short-circuiting the avalanche breakdown of the entire nanoparticle that would occur with a longer laser pulse. Instead the plasma remains confined to the region where the laser field was locally enhanced, and the ejected ions act as a pointer to the location of the nanoplasma.
The details of the location and frequency of nanoplasma formation would easily be lost if only groups of nanoparticles near the damage threshold were observed, as only the average behavior would be seen. A study of four types of nanoparticles makes it readily apparent that much more information about the underlying field enhancements can be gleaned by observing each nanoparticle in isolation, and with that understanding comes the opportunity to harness the full potential of nanotechnology.
Single nanoparticles: a study in four parts
Four aqueous solutions were used in turn to generate collimated beams containing nanoparticles roughly 100 nm in diameter. A solution of NaCl resulted in evaporation to form single crystals, while a colloidal solution of ~5-nm TiO2 nanoparticles formed 50- to 100-nm-diameter aggregate structures.
When the solution contained gold nanospheres, they appeared embedded near the surface of the nanoparticle, the remainder of which was composed of a dielectric emulsifier used in the original aqueous solution. Solutions of 50-nm gold nanospheres resulted in just one nanosphere being embedded per nanoparticle, while those formed from solutions of 17-nm gold nanospheres contained 10 or more nanospheres.
Laser intensities above 5 × 1013 W/cm2 initiated plasma formation throughout the entire nanoparticle, as expected. Reducing the laser power to approximately 3 × 1013 W/cm2 allowed the bulk material to remain unchanged, while localized plasmas formed. The threshold for complete ablation was distinct, transitioning from generation of a few ions to many very quickly with increasing laser power. Below the plasma threshold, however, each nanomaterial responded differently to the applied field, driven by uniquely different nano-optical effects.
Nanoplasma formation in the single crystals of NaCl occurred on the surface opposite the incident laser, ejecting ions in the direction of laser propagation. This is somewhat counterintuitive, yet almost every image showed field enhancement occurring after light had been transmitted through the first portion of the nanoparticle, as if experiencing subwavelength focusing (Figures 3a-d). FDTD simulations of the NaCl nanocrystals agreed, predicting a 10 percent increase in light intensity on the far side of the nanoparticle, just enough to induce localized plasma formation and explain forward ejection of ions.
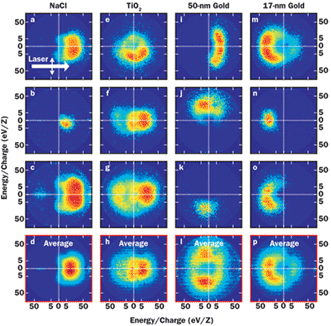
Figure 3. Photoion angular distributions from nanoparticles reveal details about the location of nanoplasma formation within nanoparticles irradiated just below the plasma threshold. (a-d) Photoions from ~100-nm NaCl crystals exhibit a nanolensing effect. (e-h) Aggregates of TiO2 crystals generate a symmetric photoion distribution. (i-l) 50-nm gold nanospheres embedded in a ~100-nm dielectric nanoparticle show random but local surface enhancement. (m-p) 17-nm gold nanospheres embedded in the same-size nanoparticle experience the greatest hot spots on the illuminated side.
The TiO2 clusters behaved quite differently, ejecting ions in all directions (Figures 3e-h). Like NaCl, TiO2 is a transparent dielectric, theoretically capable of the same subwavelength focusing effect. The difference in behavior, however, is easily explained when structure is considered, because the aggregate structure of the TiO2 nanoparticles could disrupt focusing effects. Indeed, FDTD modeling showed the greatest electric field enhancement between the TiO2 particles, with no preference for direction of laser propagation.
The nanoparticles composed of gold nanospheres in a dielectric matrix behaved differently yet again. On average, those containing just one 50-nm gold nanosphere appeared to eject ions in all directions, but each individual nanoparticle ejected ions from a different, apparently random, location (Figures 3i-l). Nanoparticles containing many 17-nm gold nanospheres showed some variation in ejected ion distribution, but the greatest density was concentrated in the direction back toward the laser (Figures 3m-p).
Again FDTD simulations helped to explain the observed behavior differences, with significant electric field enhancement on the surface of the 50-nm gold nanosphere and in the regions between the 17-nm gold nanospheres. Rapid heating of the gold relative to the dielectric matrix fuels plasma formation further.
In the presence of a single 50-nm gold nanosphere embedded in the nanoparticle, the plasma remains at the surface of the gold nanosphere, and it occurs randomly with respect to laser orientation. When many 17-nm gold nanospheres are present, modeling shows the field enhancement to be greater on the side of the nanoparticle illuminated by the laser, in agreement with the observed average behavior.
The power of one to understand many
Just as variations and similarities in the genomes of many individuals help geneticists to understand DNA, so too can the variations in behavior of individual nanoparticles in strong laser fields provide valuable insight into the mechanisms behind nanoparticle phenomena observed on a group level.
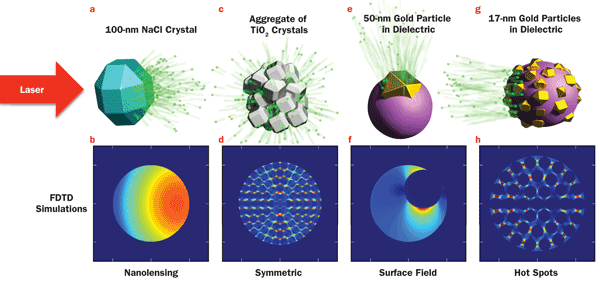
Figure 4. Localized nanoplasma formation can be explained in light of FDTD calculations of electric field intensity in the presence of a 3 × 1013-W/cm2 femtosecond laser pulse. (a) Photoion propagation in the direction of laser propagation in NaCl nanocrystals is the result of (b) a 10 percent enhancement of the laser field on the side opposite illumination. (c) Photoion ejection in all directions from a TiO2 aggregate occurs due to (d) the enhanced electric field in the regions between crystals. (e) The direction of photoion ejection is dependent on the location of a 50-nm gold nanosphere embedded within a dielectric matrix when (f) field enhancement occurs at the surface of the gold nanosphere. (g) Photoion ejection back toward the laser from a particle containing 17-nm gold nanospheres embedded in a dielectric particle is explained by the presence of (h) electric field hot spots between the gold nanospheres, which are more intense on the illumination side.
Probing nanoparticles near the plasma threshold is particularly interesting because of the novel electric field enhancement effects that can be induced by composition and structure, and its agreement with FDTD modeling. Understanding how to control nanoparticles in intense light fields is the first step toward enabling applications that harness the directionality and specificity that have been demonstrated here using just a sampling of possible materials and morphologies.
With a firm grasp on the underlying physics, it is not impossible to imagine nanolithography capable of creating features smaller than the diffraction limit, or medical therapies finely tuned to laser intensity or position. This is what an application looks like in the research stage of R&D, and it looks promising.
Meet the authors
Cicely Rathmell is a technical writer and owner at Mapleseed LLC in Dunedin, Fla.; email: [email protected]. Daniel D. Hickstein is a research fellow at JILA, a joint institute of NIST and the University of Colorado Boulder; email: [email protected]. Craig Hanson
is a product line manager at CVI Laser Optics in Albuquerque, N.M.; email: [email protected].
References
1. D.D. Hickstein et al (2014). Mapping nanoscale absorption of femtosecond laser pulses using plasma explosion imaging. ACS Nano, Vol. 8, pp. 8810-8818.
2. V.K. Pustovalov et al (2008). Photothermal and accompanied phenomena of selective nanophotothermolysis with gold nanoparticles and laser pulses. Laser Phys Lett, Vol. 5, pp. 775-792.
3. R. Lachaine et al (2014). From thermo- to plasma-mediated ultrafast laser-induced plasmonic nanobubbles. ACS Photonics, Vol. 1, pp. 331-336.
4. C.B. Schaffer et al (2001). Micromachining bulk glass by use of femtosecond laser pulses with nanojoule energy. Opt Lett, Vol. 26, pp. 93-95.
5. T.M. Antonsen et al (2005). Resonant heating of a cluster plasma by intense laser light. Phys Plasmas, 12, 056703.
6. M. Lezius and S. Dobosz (1997). Hot nanoplasmas from intense laser irradiation of argon clusters. J Phys B, Vol. 30, L251-L258.
7. T. Döppner et al (2010). Steplike intensity threshold behavior of extreme ionization in laser-driven xenon clusters. Phys Rev Lett, Vol. 105, 053401.