Dr. Wolfgang Becker, Becker & Hickl GmbH
er the past decade, confocal and multiphoton laser scanning microscopy have become
standard for biomedical imaging on the cellular level. Because these techniques
either suppress or don’t generate out-of-focus light, they produce clear images
of the selected sample plane and a near-ideal signal-to-noise ratio for a given
number of detected photons. Three-dimensional images of the sample can be obtained
by recording a stack of images in subsequent focal planes.
In recent years, scientists have used fluorescence
lifetime imaging microscopy (FLIM) and multispectral detection with laser scanning
microscopy. Multispectral detection can separate the fluorescence signals of various
fluorescent species, and FLIM can separate the signals of a particular species in
various states of interaction with the local environment.
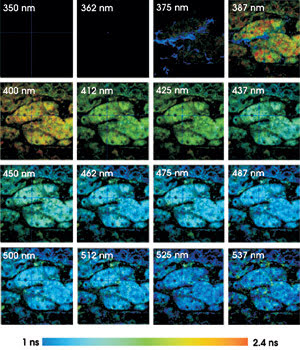
Fluorescence lifetime images of mouse kidney
tissue were taken using two-photon excitation at 750 nm, in 16 wavelength channels
from 350 to 537 nm. The lifetime from 1 to 2.4 ns is displayed by color.
FLIM-based Förster resonance energy
transfer (FRET) allows the resolution of interacting and noninteracting fractions
of labeled proteins. The technique also can extract information from autofluorescence
images and can provide quantitative results for protein-protein interactions.
For a multispectral FLIM technique,
it is important to avoid any time gating, time scanning or wavelength scanning. Gating,
both in time and in wavelength, would result in losing most of the photons. This
would require increasing the excitation power or acquisition time, which, in turn,
would substantially increase photobleaching. Photobleaching not only changes the
fluorescence lifetimes of the fluorophores, but also causes distortion in the scanned
time or in the wavelength profiles.
Moreover, multispectral FLIM should
be able to resolve the components of the complex decay functions that are typical
of autofluorescence and FRET applications.
Multidimensional time-correlated single-photon
counting solves these problems in an almost ideal way.1 The sample is excited by
a laser that delivers picosecond or femtosecond pulses at a repetition rate
of 10 to 100 MHz. The emission light is split into a spectrum by a polychromator
and is projected on the photocathode of a multianode photomultiplier tube, which
delivers an easily detectable pulse for each photon.
The time-correlated single-photon counting
module uses these pulses to construct a photon distribution over the laser pulse
period. It also records the number of the photomultiplier tube channels (colors)
that detected the photon and the coordinates of the scan. The result is an array
of pixels, each of which contains a number of spectral channels. The spectral channels
comprise a large number of time channels, each containing photon numbers for various
times over the fluorescence decay period.
Any scan rate
The technique works at any scan rate, and the
photon count rate does not have to be higher than the scan rate. The data acquisition
runs over as many frames of the scan as necessary to obtain a satisfactory signal-to-noise
ratio. The time-resolution is limited only by the transit-time spread in the photomultiplier
tube. With the currently available multianode tubes, the width of the instrument-response
function is about 150 ps (full width at half maximum). This is short enough to resolve
fluorescence lifetimes down to 100 ps without noticeable loss in accuracy. Instrument-response
function widths down to 30 ps can, in principle, be obtained with multichannel-plate
photomultiplier tubes or arrays of single-photon avalanche photodiodes.
Christoph Biskup at the Institute of
Physiology II, Friedrich Schiller University, in Jena, Germany, took autofluorescence
lifetime images of mouse kidney tissue in 16 wavelength intervals, from 350 to 537
nm (see figure). The color represents the fluorescence lifetime, and the brightness
represents the number of photons per pixel. To keep the brightness within the printable
range, all fluorescence images were normalized to 100 percent for the brightest
pixel.
The lifetime systematically decreases
with increasing detection wavelength. The signal in channel 3 (around 375 nm) is
dominated by second-harmonic generation in the tissue. Second-harmonic generation
is an extremely fast process, as can be seen in the corresponding pixels displayed
with a “decay time” shorter than 50 ps.
The data was recorded by a multispectral
FLIM system from Becker & Hickl of Berlin, connected to an LSM 510 NLO multiphoton
microscope from Zeiss with an excitation wavelength of 750 nm. The acquisition time
was 100 s and the count rate about 105 s—1. The FLIM system can process count rates
as high as 5 x 106 s—1 and thus reduce the acquisition time to a few seconds. However,
for the samples considered, the laser power that is required to obtain count rates
higher than 105 s—1 causes unacceptable photobleaching and lifetime artifacts.
Data analysis delivers lifetime images
of single- and double-exponential decay parameters from data in selected wavelength
intervals or single-wavelength channels. FLIM-FRET results can be obtained from
the double-exponential lifetime analysis of a single donor image. Even in this conventional
approach, multiwavelength FLIM data makes it easier to separate the donor fluorescence
from acceptor fluorescence and autofluorescence.
Meet the author
Wolfgang Becker is president of Becker & Hickl
GmbH in Berlin; e-mail: [email protected].
References
1. W. Becker (2005). Advanced Time-Correlated
Single-Photon Counting Techniques. Springer Series in Chemical Physics, Vol.
81. Springer.