Julien Klein and Dr. Philip G. Smith, Spectra-Physics
Recent evolutions of ultrafast laser technology uniquely overcome key challenges in bioimaging, especially for long-term in vivo imaging, deep penetration and leveraging multimodal imaging strategies.
Since the early 1990s – and even more so in the past decade – multiphoton excited fluorescence (MPEF) microscopy has seen rapid growth and wide adoption as the in vivo imaging technique of choice for various areas of biological research.1,2 Today there are thousands of commercial and homemade multiphoton microscopes, all powered by femtosecond ultrafast laser systems. Developments in ultrafast laser technology have meaningfully and, in some cases, critically enabled the ubiquitous adoption and success of MPEF microscopy in thousands of laboratories and imaging centers worldwide.
MPEF microscopy offers unique advantages for in vivo imaging. Because of the nonlinear dependence of the fluorescence signal with the intensity of the excitation light, it ensures three-dimensional resolution down to a femtoliter volume, reduces photodamage in the out-of-focus regions and allows for extended penetration depth beyond 1 mm in scattering live tissue.
Next-generation ultrafast lasers are fueling the next wave of innovation and discovery in the multiphoton imaging community. These lasers provide easy and dependable access to the long excitation wavelengths and the high-peak-power levels necessary to perform in vivo deep imaging and photostimulation for neuroscience and optogenetics, immunology and cancer research. Leveraging the high peak power delivered by the latest ultrafast laser platforms, MPEF microscopes can now perform sustained imaging of fragile live samples, such as mouse cortex, or entire organisms, such as zebra fish or C. elegans, over many hours.
Historically, the technology of choice for MPEF microscopy has been Ti:sapphire-based ultrafast lasers, with robust and dependable operation at short visible and NIR wavelengths, covering only 700 to 1000 nm. More recently, the spectral “sweet spot” for MPEF has shifted toward longer wavelengths (900-1300 nm) to improve penetration depth (through reduced optical scattering at longer wavelengths), to enhance image brightness and to reduce phototoxicity.3
The most popular fluorescent proteins are optimally excited in the two-photon regime at wavelengths above 900 nm: eGFP (930 nm), eYFP (960 nm) and red-shifted proteins such as mCherry, tdTomato, DsRed and E2-Crimson (all best excited between 1 and 1.1 µm) exemplify this trend. Moreover, new label-free techniques complementing MPEF, such as second-harmonic generation (SHG), third-harmonic generation (THG), coherent anti-Stokes Raman scattering and stimulated Raman scattering, all benefit from longer wavelengths in the NIR.
Such label-free modalities can reveal endogenous structures naturally present in biological samples to provide a more complete picture when associated with conventional MPEF. For example, SHG targets structural proteins such as collagen, while THG is sensitive to the interface of cell membranes. Coherent anti-Stokes Raman scattering and stimulated Raman scattering target lipids, while hyperspectral resonant Raman techniques have been proposed to selectively image chemical structures exhibiting specific Raman signatures in the fingerprint region.4
Pushing this even further, biologists routinely now perform multimodal imaging by combining several fluorescent proteins into one sample (e.g., GFP and mCherry), leveraging the autofluorescence of endogenous elements naturally present in tissue (e.g., NADH), or using one or several of the above-mentioned label-free techniques.
In the important and dynamic neuroscience field, the optical toolbox is rapidly expanding, and ultrafast lasers increasingly are used to optically manipulate and observe the activity of neuron networks. For example, scientists can genetically label specific classes of neurons with fluorescent proteins for imaging and localization. In the promising field of optogenetics, neurons also can be manipulated to express photoactivated channelrhodopsins (ChR2, C1V1, ReaChR). In turn, these channelrhodopsins can be precisely activated by NIR ultrafast laser pulses to trigger neuronal activity. Finally, the neuron’s electrical signals can be monitored and quantified with calcium flow as a proxy (GCaMP, RCaMP, R-GECO1)5 and now with direct voltage indicators. All three tasks (localization, activation and recording) may be performed simultaneously, and all three potentially benefit from using NIR ultrafast lasers to achieve greater penetration depth, reduced phototoxicity and more localized fluorescence generation.
Given the remarkably symbiotic relationship between ultrafast laser technology and MPEF microscopy, it is no surprise that the recent application push toward longer excitation wavelengths has coincided with a technology shift from legacy platforms to next-generation tools. By anticipating and accompanying these trends, new ultrafast laser platforms, such as the InSight DS+, have been adopted at biology laboratories and imaging centers. These new lasers are being used alongside legacy Ti:sapphire lasers and, increasingly, are replacing them.
Figure 1. Legacy Ti:sapphire platforms and their optical parametric oscillator extensions deliver limited power above 900 nm, where most of today’s multiphoton excited fluorescence imaging takes place. New platforms such as InSight DS+ are optimized for high peak power above 900 nm to drive much increased fluorescence and deeper penetration, as is illustrated by the shaded area. Courtesy of Spectra-Physics.
Consider Figure 1. At shorter wavelengths, below 900 nm, Ti:sapphire platforms historically have delivered impressive average (>3 W) and peak powers (>400 kW). In most cases, these power levels are excessive and require significant external attenuation to preserve biological samples. However, between 900 and 1000 nm, the power available from Ti:sapphire drops rapidly, and above 1000 nm, power levels are marginal, especially for deep in vivo imaging. In contrast, the technology behind InSight DS+ was developed with one focus: to generate high peak power (up to 200 kW) at the right wavelengths above 900 nm. This platform constitutes the optimal tunable excitation source for GFP, YFP and RFP. In the 1- to 1.3-µm spectral region, the laser delivers two times more peak power than Ti:sapphire-pumped optical parametric oscillators, promising the deepest possible penetration in live tissue when used in conjunction with an appropriate red-shifted fluorophore. This peak power advantage is best appreciated when referring to the shaded area in Figure 1.
By incorporating dual-output beams to enable simultaneous excitation, new platforms are ready for the ever-changing needs of multimodal imaging. This flexibility proves extremely useful in a number of cases.
Dr. Marie Irondelle of the Institut Curie/CNRS in Paris studies tumor development in the mammary gland (Figure 2).

Figure 2. SHG and THG label-free imaging reveals structural details of a mouse mammary gland, including collagen (in magenta) and adipocytes (in yellow). Data was taken using a 1250-nm excitation wavelength from an InSight DS+ tunable beam. Courtesy of Dr. Marie Irondelle.
In this ex vivo experiment, a mouse mammary gland was imaged in a label-free scheme. With a 1250-nm wavelength, Irondelle simultaneously observed collagen with SHG imaging (in magenta) and adipocytes with THG imaging (in yellow). Remarkably, this image was generated without any external fluorescent proteins, relying on label-free modalities.
Multimodal imaging now can be performed in vivo over long periods of time. Dr. Michael Kuligowski of the Centenary Institute of Cancer Medicine and Cell Biology in Sydney investigates the interaction between T cells (white blood cells that play a central role in cell-mediated immunity) and dendritic cells in the skin (Figure 3). Here, T cells labeled with CMTPX (a red-shifted fluorescent dye), were taken from a donor mouse and injected in another mouse with dendritic cells labeled with GFP. The GFP-labeled dendritic cells were imaged using a Ti:sapphire laser (the Mai Tai DeepSee) tuned to 925 nm, and the CMTPX-labeled T cells were imaged with the InSight DS+ tuned to 1080 nm. The interaction was observed over several hours, and the snapshots shown in Figure 3 follow a single T cell (marked with a white arrow) interacting with a dendritic cell for approximately 40 minutes. Each image originated from a 300-µm Z-stack with a 411 × 411-µm field of view.

Figure 3. Two-color simultaneous imaging allows for the continuous tracking of T cells (tagged with CMTPX, imaged at 1080 nm) and dendritic cells (tagged with GFP, imaged at 925 nm) as they interact in mouse skin over several hours. Elapsed times are listed in the upper left corner in hh:mm:ss format. Courtesy of Dr. Michael Kuligowski, Centenary Institute of Cancer Medicine and Cell Biology.
This type of long-term monitoring and tracking now can be extended to full organisms; for example, to the zebra fish (Danio rerio) model. The early development of a whole zebra fish embryo can be continuously imaged using MPEF microscopy at reduced average power levels (i.e., tens of milliwatts). In this example, Dr. Nadine Peyriéras of the French National Center for Scientific Research (CNRS) in Gif-sur-Yvette, France, tracked development over eight hours using a microscope provided by LaVision BioTec and equipped with the InSight DS+ Dual (Figure 4). The embryo cell membranes were labeled with eGFP, and the cell nuclei with mCherry. The eGFP and mCherry were imaged simultaneously at 980 and 1041 nm, respectively, using the laser’s dual-beam configuration. The embryo was imaged from both front and back sides sequentially. Each image is a maximum-intensity projection created from a 130-frame Z-stack. The eight-hour movie depicts the embryo development in impressive detail, as is illustrated by the four snapshots taken at approximately time 0, 4, 6 and 8 hours.
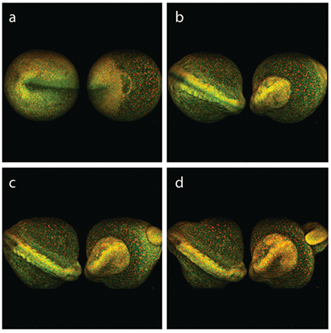
Figure 4. Multiphoton excited fluorescence imaging is compatible with long-term monitoring of live zebra fish embryo development. Simultaneous tracking of cell membranes (tagged with eGFP, imaged at 980 nm) and cell nuclei (tagged with mCherry, imaged at 1041 nm) reveals the development with impressive details. Embryo development at (a) 0, (b) 4, (c) 6 and (d) 8 h. Courtesy of Dr. Nadine Peyriéras, CNRS, and LaVision BioTec.
Meet the authors
Julien Klein is senior manager of product marketing for bioimaging applications at Spectra-Physics in Santa Clara, Calif.; email: [email protected]. Dr. Philip G. Smith is the senior product marketing manager for ultrafast oscillators at Spectra-Physics in Santa Clara, Calif.; email: [email protected].
References
1. W. Denk et al (1990). Two-photon laser scanning fluorescence microscopy. Science, Vol. 248, pp. 73-76 (doi: 10.1126/science.2321027).
2. A. Krueger (2010). Multiphoton microscopy: Turnkey femtosecond lasers fuel growth of multiphoton imaging. Laser Focus World, Vol. 46, pp. 39-43.
3. D. Kobat et al (2009). Deep tissue multiphoton microscopy using longer wavelength excitation. Opt Express, Vol. 17, pp. 13354-13364 (doi: 10.1364/OE.17.013354).
4. D. Fu et al (2013). Hyperspectral imaging with stimulated Raman scattering by chirped femtosecond lasers. J Phys Chem B, Vol. 117, p. 4634 (doi: 10.1021/jp308938t).
5. J. Akerboom et al (March 4, 2013). Genetically encoded calcium indicators for multi-color neural activity imaging and combination with optogenetics. Front Mol Neurosci (doi: 10.3389/fnmol.2013.00002)