Niten Olofsson, Iakovos Lazaridis, Konstantinos Meletis and Marie Carlén, Karolinska Institutet, and Ulf Tingström and Håkan Karlsson, Cobolt SE
A guide to suitable light sources and fiber optic components for optogenetics research, with a specific emphasis on rodent behavior.
The use of lasers and optics for optogenetics is only in its infancy. In the next decade, we can expect researchers to uncover many inner secrets of the human brain and possibly also find cures and treatments for some of our most challenging psychiatric disorders and diseases, helping us to a better life.
Optogenetics builds on the discovery that light-activated molecules called opsins can be expressed in neurons (Figure 1). Light-sensitive neurons can be manipulated with light with a very temporal and spatial precision at timescales relevant to the brain’s normal processing. The engineering of opsins used for optogenetics is an exploding area in itself, with many new and drastically modified opsins steadily becoming available to meet the needs of research applications, including those relevant to monkeys and humans.
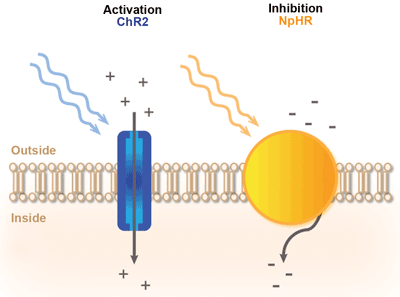
Figure 1. Principle of light-mediated control of neural activity. The commonly used activating opsin ChR2 allows entry of positively charged ions upon illumination with blue light. As a result, the neuron’s membrane is depolarized, and action potentials are generated. The inhibiting opsin NpHR pumps negatively charged ions into neurons upon illumination with yellow light. As a result, the neuron’s membrane is hyperpolarized, and action potential initiation is inhibited. Adopted from Reference 6.
The fast-moving, multidisciplinary nature of optogenetics represents an important challenge. In particular, it is a demanding task for many researchers to determine an adequate set of light sources and fiber optic components for their specific optogenetics application. To address the need for user-friendly laser assemblies, Cobolt AB has worked with the laboratories of Marie Carlén and Konstantinos Meletis at Karolinska Institutet in Stockholm to develop a series of laser tools.
The optogenetics setup
For optogenetic manipulations in live animals, the light sources used are almost exclusively lasers. Lasers produce a beam of almost parallel light with very low divergence, allowing it to be efficiently focused into an optical fiber – typically with a core diameter of 50 to 300 μm. Many optogenetics experiments can be conducted using one opsin and unilateral optical manipulation of the cells of interest (i.e., in one hemisphere).
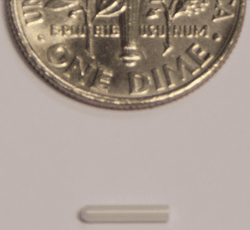
Figure 2. Ferrule used for implantation of optical fiber into the brain.
Three different configurations have been carefully designed to meet the requirements of optogenetics experiments: single-line lasers with a fiber manipulator; two lasers on a common platform launched into one common fiber manipulator; or two lasers sitting side by side launched into one manipulator each, suitable for a two-into-one combination with a fused fiber. The lasers in the assemblies are available with 100 mW of adjustable output power at the wavelengths 473, 561, 594 and 638 nm, where the 561 and 594 nm are diode-pumped solid-state (DPSS) lasers with modulators or shutters.
Parameters to consider include the fiber core diameter and how to insert the fiber into the brain. Preassembled implantable sleeves holding a short fiber are available (Figure 2). During the experiment, a longer fiber is inserted into the sleeve, requiring perfect surface contact between the short and long fiber. Alternatively, a cannula guide can be implanted and a continuous, longer fiber inserted through the cannula into the brain during the experiment. The end of the fiber is coupled to a connector that is compatible with the connector on the laser assembly’s manipulator (e.g., SMA or FC).
More complex experiments can require the application of light of two separate wavelengths, bilateral stimulation (both hemispheres) and the animal’s free movement. Two main strategies are currently used to deliver two different wavelengths. In the first, the two light beams are directed and combined into one fiber manipulator using free-space optics. A single fiber is coupled to the laser assembly but can be split into two fibers, if needed. The second strategy avoids the use of free-space optics, and the two light beams are directed to one fiber manipulator each. Two fibers are then connected to the laser assembly and can be fused into one fiber, if needed. To allow for free movement of the animal and to avoid twisting and turning the fiber(s), a rotary joint can be added (Figure 3).
Figure 3. A laser assembly with connected optical fiber with split fiber ends and a rotary joint.
Lasers for optogenetics
It is a true challenge for neuroscientists to achieve sufficient light exposure in an area of the brain without overexposing tissue and creating damage. At the target site, a power density of 1 to 10 mW/mm2 is typically needed for successful manipulation of neuronal activity, but opsins with increased light sensitivity are constantly being engineered to reduce the amount of light needed. The selection of a laser source for optogenetics is quite delicate, and a few parameters must be considered with care.
First, after an appropriate opsin is selected, a laser with an output wavelength matching the sensitivity of the opsin must be chosen. Currently, the most commonly used wavelengths are 473, 532, 561, 594 and 638 nm.
Figure 4. Typical pulse fidelity graph for a Cobolt MLD 473-nm laser with direct modulation.
Second, the laser must provide sufficient output power for successful activation of the opsin. Thus, the laser must have power headroom for all the losses in the fiber delivery system. The power also needs to be adjustable, as the light requirements for generating photocurrents can vary considerably from one animal to another because of varying degrees of expression of the opsin, for example. Adjustable power is also needed to avoid tissue damage from excessive light exposure. A typical need for rated power is at least 100 mW, with a useful power range from below 20 mW up to a rated maximum.
The third important parameter is the power stability over time. This is crucial, as an experiment can run for hours, and stable power ensures that artifacts are not created from variations in the light application. Therefore, power variation of less than 2 percent is a must.
The laser’s modulation capability must also be considered. The frequency of modulation is dictated by the physiological process the optogenetic manipulation intends to replicate or model; it is influenced by the opsin’s kinetic properties. Some activity patterns in the brain are slow and on the order of seconds, while others reach over 100 Hz.
Figure 5. Typical pulse fidelity graph for a Cobolt Mambo 594-nm laser with built-in modulator.
It is also very important that the rise/fall times of the light pulse are short, accurate and on the millisecond scale. It is equally important that the pulse shape and the power level from one pulse to another are consistent and repeatable throughout the experiment.
The two main types of lasers used for optogenetics are DPSS lasers and laser diodes. Both compact in design and power efficient, they do not require any cooling systems. Laser diodes, the most cost-effective solution, are available in blue and red wavelengths, although not yet in green, yellow or orange. They can be directly modulated with great accuracy and speed, limited only by the electronic drive circuitry (Figure 4). Laser diodes can be set at powers of close to zero or up to their maximum rated power with no difference in stability.
DPSS lasers are powerful, high-performance devices commonly used in many demanding applications. They are available in many wavelengths across the visible spectrum, with 532, 561 and 594 nm typically used in optogenetics. All optically pumped lasers, such as DPSS lasers, are inherently limited by their construction where a diode laser is pumping a laser crystal inside a laser cavity. The optically pumped lasers will run stable only at continuous operation and at maximum rated power, unless carefully engineered to function differently.
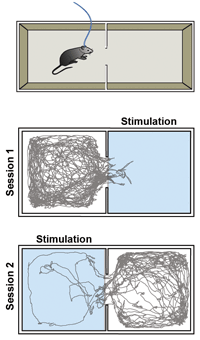
Figure 6. This model demonstrates real-time place preference. In two consecutive 10-min sessions with 20 seconds in between, laser stimulation is first triggered by the mouse’s entrance into the right chamber, and thereafter into the left chamber. The second session is started while the animal is still in the left chamber, as can be seen from the trace. A Cobolt MLD 473-nm laser with maximum output power of 120 mW was used with a branching patch cord from Doric lenses. A microcontroller, Arduino, was programmed to control the laser, and Biobserve software tracked the position of the mouse. The stimulation rate was 60 Hz, with 2-ms pulse length.
DPSS lasers can be designed for a wide dynamic power range and also with limited modulation capability. However, directly modulated DPSS lasers typically do not have good enough pulse-to-pulse consistency and power stability during modulation to meet the demands of optogenetic applications. Therefore, DPSS lasers used for optogenetics need a built-in modulator or a fast shutter to modulate the light, allowing the devices to operate continuously and hence with as much stability as the design allows (Figure 5).
Sample experiment: real-time place preference
In this experiment, a population of neurons involved in aversive behavior – i.e., responsible for driving a behavior where unpleasant situations are avoided – is probed. The blue-light-sensitive activating opsin ChR2 is targeted to the neurons of interest in one hemisphere of a mouse’s brain, and a single 200-µm optical fiber is implanted with the fiber tip placed directly adjacent to the neurons to be manipulated. A rectangular box divided into two chambers by a wall holding an open passage is used to probe the behavior. Tracking software detects the mouse’s movement, and the mouse’s entrance into one of the chambers triggers activation of the blue laser, resulting in activation of the neurons of interest.
Figure 7. Normalized activation spectra for a selection of opsins. ChR2(H134R),10 Chronos,11 C1V1TT10 and Chrimson11 are all activating opsins derived from either channelrhodopsin-1 or -2. eNpHR3.012 is a chloride pump derived from halorhodopsin. Data is extracted from referenced articles.
Figure 6 shows that application of light leads to direct expression of aversive behavior, as the mouse completely avoids the chamber where the laser is activated. To certify that place aversion is driven by activation of the neurons of interest and not by external factors, the laser is subsequently triggered in the other chamber. As a result, the mouse now avoids this chamber.
Meet the authors
Niten Olofsson, Iakovos Lazaridis, Konstantinos Meletis and Marie Carlén are optogenetics researchers in the department of neuroscience at Karolinska Institutet in Stockholm. Ulf Tingström is director of marketing and sales at Cobolt SE; email: [email protected]. Håkan Karlsson is Cobolt’s CEO; email: [email protected].
Figure 8. Kinetics (τoff) and spectral parameters (λ) for a selection of opsins. In the planning of optogenetics experiments requiring activation of two separate neuronal cell populations or concurrent activation and inhibition of one neuronal population, the activation wavelengths are compared. The opsin’s decay kinetics must be considered in experiments involving pulsing of light at defined frequencies. Activating opsins (white circles): ChR2(H134R),5 C1V1TT (= C1V1 ChETA (E122T/E162T)),5 ChETA (= ChR2(E123T)),5 Chrimson,11 Chronos,11 ChIEF510 and SSFO (= ChR2(C128S/D156A)).5 Inhibiting opsins (black squares): eNpHR3.0,5 NpHR,5 Jaws,12 ArchT1212 and iC1C2.9 Data is extracted from referenced articles. Adopted and extended from Reference 5.
References
1. F.H. Crick (1979). Thinking about the brain. Sci Am, Vol. 241, pp. 219-232.
2. G. Nagel et al (2005). Light activation of channelrhodopsin-2 in excitable cells of Caenorhabditis elegans triggers rapid behavioral responses. Curr Biol, pp. 2279-2284.
3. F. Zhang et al (2007). Multimodal fast optical interrogation of neural circuitry. Nature, Vol. 446, pp. 633-639.
4. K.M. Tye and K. Deisseroth (2012). Optogenetic investigation of neural circuits underlying brain disease in animal models. Nat Rev Neurosci, Vol. 13, pp. 251-266.
5. O. Yizhar et al (2011). Optogenetics in neural systems. Neuron, Vol. 71, pp. 9-34.
6. F. Zhang et al (2007). Circuit-breakers: optical technologies for probing neural signals and systems. Nat Rev Neurosci, Vol. 8, pp. 577-581.
7. O. Yizhar et al (2011). Neocortical excitation/inhibition balance in information processing and social dysfunction. Nature, Vol. 477, pp. 171-178.
8. J.Y. Lin et al (2013). ReaChR: a red-shifted variant of channelrhodopsin enables deep transcranial optogenetic excitation. Nat Neurosci, Vol. 16, pp. 1499-1508.
9. A. Berndt et al (2014). Structure-guided transformation of channelrhodopsin into a light-activated chloride channel. Science, Vol. 344, pp. 420-424.
10. J. Mattis et al (2012). Principles for applying optogenetic tools derived from direct comparative analysis of microbial opsins. Nat Methods, Vol. 9, pp. 159-172.
11. N.C. Klapoetke et al (2014). Independent optical excitation of distinct neural populations. Nat Methods, Vol. 11, pp. 338-346.
12. A.S. Chuong et al (2014). Noninvasive optical inhibition with a red-shifted microbial rhodopsin. Nat Neurosci, Vol. 17, pp. 1123-1129.
A brief history of optogenetics
The history of the concept of optogenetics dates back to the 1970s, when Dr. Francis Crick, co-discoverer of DNA, put to words that neuroscience needed a method to control one type of nerve cell while leaving the other cells unaffected, to understand how the brain works.1 Crick later also suggested that light would be the means to control the neurons from the outside, allowing arbitrary timing precision at timescales relevant to the brain’s processing.
Independently, biologists at the time had identified microorganisms capable of regulating the flow of ions (electric charge) through their membranes with the aid of light-sensitive proteins (opsins). Building on the fact that the activity of neurons is regulated by the flow of ions through the plasma membrane, scientists in the late 1990s started exploring the use of light-sensitive proteins for controlling neurons, but researchers did not recognize opsins as being suitable for neuroscience until the 2000s. A combined effort by several research groups bore fruit in 2005 when, for the first time, light plus a single-component light-sensitive ion channel was used to activate neurons and change the behavior of a living animal.2 Light-activated proteins that could inhibit the activity of neurons were soon after identified, and in 2007 the first use of an inhibiting opsin for control of behavior was demonstrated.3
Since 2005, when the expression was coined and the technology introduced, optogenetics has found many applications and is regarded as one of the biggest revolutions in the study of the brain and its functions. The ultimate frontier of neuroscience is understanding how the brain gives rise to our behavior, and optogenetics has already proved its unprecedented utility. Activating and inhibiting opsins have been targeted to a variety of types of neurons and brain areas in rodents to identify cells and regions in the brain central to fear, anxiety, sleep, hunger, social behavior, learning, memory, aggression, motivation and more. In addition, optogenetics has been widely used for understanding how changes in brain activity can manifest themselves as disorders such as depression, schizophrenia, autism, Parkinson’s disease, epilepsy and drug addiction, to mention a few.4
But behavior does not constitute the only readout of optogenetic manipulations, and much can be learned about the brain by combining optogenetics with other technologies, such as electrophysiology or imaging.
Opsins can be categorized by their effect (activation vs. inhibition of neuronal activity), spectral sensitivity (peak and interval wavelengths), means of ion transport over the cell membrane (channel or pumps), on and off kinetics, photocurrent properties and more. Most opsins can be activated by a wide interval of wavelengths (Figures 7 and 8). At the peak interval, maximum activation and photocurrent are generated. The currently available light-activated proteins used for optogenetics have a peak excitation wavelength somewhere in the visible or infrared spectrum.5 The most commonly used activating opsin, Channelrhodopsin-2 (ChR2), has a peak activation around 470 nm (blue light), and the opsins most commonly used for inhibiting neuronal activity (halorhodopsins, NpHRs) are optimally activated by yellow light (peak activation around 590 nm).
Importantly, in recent years a vast flora of opsins has been engineered for optogenetics. As the need for concurrent manipulations has been realized, opsins have been developed that, for example, allow interchangeable activation and inhibition of a population of neurons,6 or parallel activation of two different populations of neurons within the same brain area,7 both achieved through application of light of different wavelengths. Crucial in these examples is the ability of the different wavelengths of light to selectively activate one opsin while leaving the other opsin unaffected. If two opsins are to be targeted to the same brain area, the spectral overlaps and the relative strengths of the photocurrents generated must be taken into account when choosing stimulation wavelengths and power densities.
Additional recent advances in optogenetics include the development of opsins that are activated by one wavelength of light and inactivated by a different wavelength – the so-called step-function opsins.5 A single light pulse of one wavelength activates the opsin and triggers a gradual change in the ion flow over a neuron’s membrane that can be terminated by a light pulse of a different wavelength. Most commonly, blue light is used for activation and red light for inactivation, but other combinations have also been used. There have long been efforts to engineer activating opsins sensitive to red light.8
Fiber implantation requires complex and high-precision microsurgery, and often creates a certain degree of brain damage. As blue and green wavelengths scatter in the brain and are absorbed by blood, the fiber tip must be placed in the direct vicinity of the neurons under investigation for effective activation of the opsins, making it difficult to target small and/or deep brain structures. Red light penetrates brain tissue more effectively, displays less scattering and absorption in blood, and can even activate neurons through an intact skull.8 An important and recent development has been the generation of an inhibiting channelrhodopsin, sensitive to blue light.9 This new tool enables fast and more physiological optical inhibition of neuronal activity and displays increased light sensitivity.