How to Make a Laser Beam Without a Laser Resonator
Amplified spontaneous emission produces a well-collimated output beam.
Breck Hitz
Superradiant lasers — ones that produce enough gain in a single pass to generate significant output — have been around since the early days of nitrogen lasers in the 1960s. Recently, scientists at Imperial College London refined the concept to produce a resonatorless Nd:YVO4 laser that generates 30 W at 1.06 μm in a very highly collimated — M2 ~1.3 — beam. The advantage of a resonatorless laser is that it has a high tolerance to component misalignment and other perturbations.
In a conventional laser, mirrors form a resonator in which light at certain specific frequencies oscillates. The light passes multiple times through the gain medium, and an output coupler — usually one of the mirrors — extracts a small amount of the circulating power. Photons in the emerging beam have been traveling in a collimated beam for many round-trips inside the resonator; hence, they remain well-collimated in the output beam.
In a resonatorless laser, on the other hand, light at any frequency builds up by amplified spontaneous emission and makes only one or, at most, several passes through the gain medium. Because the emerging photons have been traveling together for a relatively short distance, they are usually less well-collimated than the photons emerging from a conventional laser. The London scientists extended the length of the gain medium so that the photons emerging from their laser had been traveling together over a relatively long distance and, hence, were well-collimated.
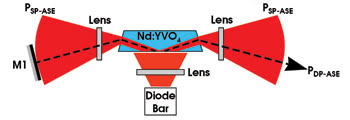
Figure 1. The scientists operated their single-slab amplified spontaneous emission laser in both a single-pass configuration (SP, without mirror M1), and in a double-pass configuration (DP, with the mirror). Images reprinted with permission of Optics Letters.
The researchers started with a simple configuration of their resonatorless laser (Figure 1). They side-pumped a rectangular slab of Nd:YVO4 with an 808-nm diode bar. When mirror M1 was not present, uncollimated amplified spontaneous emission emerged in a wide cone (the red cone in Figure 1). The laser produced a total of only 158 mW in both directions from 35 W of pump power.
When the scientists inserted mirror M1 into the configuration, it reflected only part (dotted line in Figure 1) of the amplified spontaneous emission for a second pass through the slab. This light was refracted at the air-YVO4 interface as shown in the figure and bounced off the side of the YVO4 slab because of total internal reflection. This crooked path through the slab allowed for significant spatial averaging of both the gain and the thermal inhomogeneities in the rod. Moreover, because the crooked path increased the optical path length in the gain medium, the unsaturated gain was as high as ~104. Because a second pass through the slab extracted more energy from the population inversion, the output power at 35 W of pump power was 5.2 W, compared with 158 mW for the single-pass case.
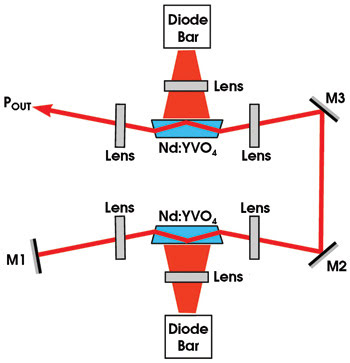
Figure 2. A second Nd:YVO4 amplifier resulted in more power in a better-collimated beam.
To extend the physical length of the gain medium and to scale up the power further, the scientists added a second Nd:YVO4 slab to the configuration (Figure 2). Pumping both slabs at 40 W, they observed 24.5 W of 1.06-μm output in a collimated — M2 ~1.2 vertical, ~2.5 horizontal — beam.
They achieved even greater collimation and power by looping the output of the second slab back for another pass through the first slab (Figure 3). In this case, with a total of 80 W of pump power, the laser generated 30 W of 1.06-μm output in a beam with horizontal M2 <1.3 and vertical M2 <1.2.
The power dynamics of the resonatorless laser were in some respects as subtle as the intracavity dynamics of a conventional laser. Thinking of the system in Figure 3 as three in-line amplified spontaneous emission amplifiers is an oversimplification because light travels in both directions through the slabs and tends to couple the slabs. One result is that weak standing waves could be formed, with spatial hole burning and gain-grating formation. The scientists plan to investigate this effect.
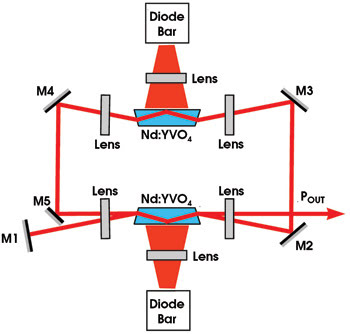
Figure 3. By looping the output from the second vanadate slab back through the first slab, the scientists created a resonatorless laser whose power and beam quality are equivalent to a conventional laser’s but whose tolerance for mirror misalignment is greater.
Another subtlety is that gain saturation in the first slab introduces a stabilizing negative feedback. If the amplified spontaneous emission in either of the slabs increases, the power looped through the first slab increases, pushing its gain closer to saturation and effectively stabilizing it, thereby compensating for the original increase in amplified spontaneous emission.
The scientists believe that they saw evidence of this behavior in the laser’s spectrum, which fluctuated between the broadband featureless spectrum expected from an amplified spontaneous emission source and the comblike spectrum expected from the longitudinal modes of a laser resonator. The modes corresponded to a parasitic oscillation between mirror M1 and the cylindrical lens on the right side of the first slab.
The researchers propose that when the gain in the first slab was relatively unsaturated, it was substantial enough to allow the parasitic oscillation. Then, as the gain saturated, the parasitic oscillation dropped below threshold and the spectrum returned to the featureless amplified spontaneous emission spectrum.
The scientists recently scaled the resonatorless laser concept to near 100 W of excellent beam quality. They believe that multihundred-watt resonatorless lasers can be built with little sacrifice of beam quality.
Optics Letters, July 1, 2007, pp. 1911-1913.
Published: September 2007