GRIN lenses help make miniature instruments for in vivo imaging.
Dr. Bernhard Messerschmidt, Grintech GmbH
Morphologic and functional imaging of living cells in deep tissue with micronscale resolution is key for understanding biological and disease processes. For example, high-resolution imaging can help reveal information about cancer generation in the brain or in the colon and can reveal the pharmacologic effects of drugs on a cellular level. However, many organs (whether in humans or in animal models of disease) are inaccessible by conventional light microscopy.
To access these organs requires small instruments that can be maneuvered inside the body. Because gradient index (GRIN) optics can have diameters between 0.25 and 2.0 mm, they can be used to create miniature microscopic instruments with resolutions down to less than 1 μm. These systems can be built with small magnifying objectives that attach to flexible fiber optics, so that a handheld flexible probe can be introduced to deep tissue regions. Alternatively, rigid tubes a few centimeters long can incorporate GRIN relay optics that transfer the microscopic image out of the subject to be recorded by conventional microscopic setups such as epifluorescence, confocal or multiphoton microscopes. Also, in optical coherence tomography (OCT), miniaturized probes use gradient index lenses to focus the scanning beam on the tissue of interest.
To understand how a GRIN lens works, it is necessary to understand how a conventional lens works. In a conventional lens, incoming light is refracted when it enters the shaped lens surface because of the abrupt change of the refractive index from air to the homogeneous material (Figure 1, right). The light passes through the lens material directly until it emerges through the exit surface, where it is refracted again because of the abrupt index change from the lens material to air. The surface shape of the lens causes the rays to focus on a spot. The high precision required for the fabrication of the surfaces of conventional lenses aggravates the miniaturization of the lenses and raises the cost of production.
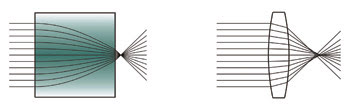
Figure 1. A gradient index (GRIN) lens (left) generates its optical power by a refractive index profile inside the medium. Plane optical surfaces allow miniaturization down to 0.25 mm and easy integration with other planar optical components. A GRIN lens does not require the refraction at curved surfaces that conventional lenses do (right).
Instead of depending on the shape of the lens surface, GRIN lenses depend on a continuous change of the refractive index within the lens material. The light rays are continuously bent by the refractive index changes within the lens until finally focusing on a spot (Figure 1, left). In contrast to conventional lenses, curved optical surfaces are not required and are replaced by plane optical surfaces, which are much easier to fabricate on small lenses.
In GRIN lenses, the focus position with respect to the surface of the lens and the focal length can be easily adjusted by cutting the lens at a different thickness along the optical axis. The plane surfaces of the lenses can directly touch the medium of interest; i.e., the tissue, water or some other index-matching medium, without sacrificing the lens performance. The focal plane position inside the object medium can vary between zero and a few hundred microns and is determined by the design of the lens thickness. Also, other types of plane optical components such as long GRIN relay lenses, spacer rods, optical fibers or prisms for changing the direction of view can be mounted by direct contacting and optical gluing. The maximum numerical aperture, which determines the maximum acceptance angle of the system that can be achieved by a single GRIN lens, equals about 0.55 and results from the refractive index change of the GRIN profile between the center and the margin of the lens.
The lenses are typically produced by ion exchange in rods of an aluminoborosilicate glass containing a large portion of monovalent ions as sodium. By immersing these glass rods in salt melts at temperatures between 300 and 600 °C, an interdiffusion process of cations in the glass and the salt melts occurs, creating the local variation of the refractive index in the lens material. Longer glass rods are later cut into smaller pieces and ground and polished to the final lens thickness. The highest numerical apertures are produced by silver-sodium ion exchange, while lower numerical apertures (less than 0.2) are obtained by lithium-potassium-sodium exchange.
The design of optical systems with GRIN lenses is very similar to conventional optics. Image quality, magnification, focal lengths, working distances, etc., are optimized by changing the lens thickness or the spaces between the optical components. However, the spaces can be homogeneous glass rods with plane optical surfaces that are directly glued between the optical components. This procedure simplifies the assembly of miniaturized optical systems dramatically because no precision-mechanical holders or mounts are needed to build up the system.
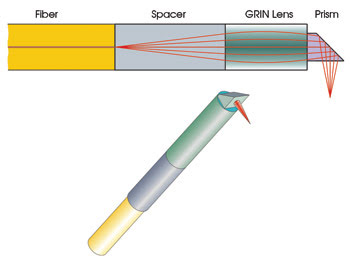
Figure 2. A typical fiber optic GRIN subassembly consists of a fiber terminated by a ferrule, a glass rod spacer for adjusting the beam diameter at the entrance of the GRIN lens, the GRIN lens itself and a microprism to deflect the beam. Standard probes range from 0.5 to 1.8 mm in diameter and are applied in endoscopic OCT setups.
OCT systems
Small probes for OCT are typical examples of fiber optic GRIN subassemblies, where a glass rod spacer is used between a fiber ferrule and the GRIN lens to control the size, divergence and position of the scanning spot. A prism is attached to the front side of the lens to deflect the scanning spot of the rotating probe to the side (Figure 2). Probes with diameters down to 0.5 mm and 90° prisms are standard, while other deflecting angles and scanning mechanisms also are being developed. Changhuei Yang’s group at California Institute of Technology in Pasadena, for example, has used a dual GRIN lens design with the lenses facing each other and the surfaces angled at 15°. The deflection angle of a spiral scan can be controlled by varying the rotational orientation between both lenses (Figure 3).1
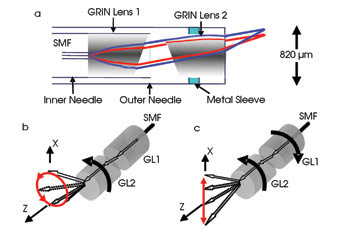
Figure 3. The endoscopic OCT probe uses paired-angle rotating scanning and consists of two angle-polished GRIN lenses (a). Holding GL1 stationary and rotating GL2 produces a circular scan (b). Rotating both GL1 and GL2 in opposite directions produces a linear scan that retraces itself after 180° (c).
In vivo imaging of deep tissue can be performed using miniaturized gradient index optical systems. Single-photon fluorescence laser scanning systems use magnifying telescope probes of two different GRIN lenses to image the tissue with a flexible coherent imaging fiber bundle. The bundle transports the image to the laser scanning acquisition system. The GRIN optics system can be directly attached to the fiber bundle, and the working distance between the object plane in the tissue and the probe entrance face can be adjusted by grinding and polishing the objective lens to the appropriate length.
The lateral resolution of this type of imaging often is limited by the separation between the individual fibers in the fiber bundle, which is typically in the range of 3 μm. However, with the magnification of a GRIN telescope system, which is often chosen to be about 2.5×, an object space resolution of about 1 μm can be obtained.
Another way to perform in vivo imaging is to access the tissue using a rigid tube containing a GRIN objective and relay optics up to a few centimeters long. The image is brought to a conventional microscope, and focusing through the GRIN optics can be done without moving the inserted tube in the vulnerable tissue. This procedure can record Z-stacks of different tissue layers.
Using this type of setup, a lateral resolution of less than 1 μm has been shown by researchers led by Mark Schnitzer at Stanford University in California. They performed one- and multiphoton fluorescence imaging using GRIN doublets and triplets with diameters between 0.35 and 1.0 mm.2 This group is successfully applying these probes to in vivo neuronal imaging and to monitoring individual blood cells in the smallest blood vessels. The researchers also are developing GRIN multiphoton microscopes that weigh only 3 g. In these systems, femtosecond laser pulses are delivered using a flexible photonic crystal fiber, and a MEMS mirror setup performs the scanning (Figure 4).3,4
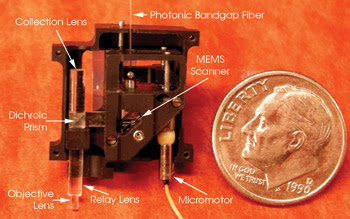
Figure 4. This photograph shows the inner components of a portable fiber optic two-photon microendoscopy device based on a MEMS scanning mirror. The mirror is microfabricated in silicon by photolithography methods and deflects light in two angular dimensions with a fast-axis scanning rate of 1.7 kHz. Ultrashort pulses of light from a Ti:sapphire laser are delivered to the instrument by a hollow-core photonic bandgap fiber that virtually eliminates ultrashort pulse distortion. The mirror scans the light in a raster pattern. The light then enters an optical assembly consisting of a dichroic beamsplitter cube and four GRIN lenses of different diameters. Two-photon-excited fluorescence is generated in the sample at the focal spot of the objective lens, passed back unreflected through the beamsplitter cube and focused by a collection lens into a multimode optical fiber (not shown) that routes to a photodetector.
Previous applications of GRIN systems in the microendoscopic field have been limited in resolution by the maximum numerical aperture of the objective lens, which is ~0.5, and partially by residual aberrations of the index profiles. The goal of ongoing development is to enhance the GRIN lens’s object side numerical aperture to 0.7 or even up to 0.85, while keeping the lenses small enough and maintaining a plane entrance window that can directly touch the tissue.
Initial prototypes of these new designs with optics 1.0 mm in diameter have been presented.5 They showed a lateral resolution improved to 0.55 μm and an axial resolution of 1.7 μm in multiphoton imaging. Similar systems are being tested in biological systems and soon will be commercially available.
Meet the author
Bernhard Messerschmidt is chief technical officer of Grintech GmbH in Jena, Germany; e-mail: [email protected].
References
1. M.V. Sarunic et al (Feb. 7, 2007). Endoscopic optical coherence tomography of the retina at 1310 nm using paired-angle rotating scanning. SPIE PROC, Vol. 6429, 642911.
2. J.C. Jung et al (2004). In vivo mammalian brain imaging using one- and two-photon fluorescence microendoscopy. J NEUROPHYSIOL, Vol. 92, pp. 3121-3133.
3. K. Deisseroth et al (Oct. 11, 2006). Next-generation optical technologies for illuminating genetically targeted brain circuits. J NEUROSCIENCE, 10380-10386.
4. W. Piyawattanametha et al (2006). Fast-scanning two-photon fluorescence imaging based on a microelectromechanical systems two-dimensional scanning mirror. OPT LETT, Vol. 31, pp. 2018-2020.
5. B. Messerschmidt et al (2007). Novel concept of GRIN optical systems for high resolution microendoscopy: Part 1. Physical aspects. SPIE PROC, Vol. 6432, 643202.