This increasingly popular research tool is opening the way to structures beyond the reach of traditional optical microscopes.
RUSSELL ULBRICH, OLYMPUS AMERICA INC., SCIENTIFIC SOLUTIONS GROUP
Much of our understanding of biology has come from our ability to image biological processes and structures through microscopes. But until recently, the imaging of intracellular structures and activities has been constrained by the optical resolution limit of the imaging systems themselves. This diffraction limit, first stipulated by Ernst Abbe in 1873, established the maximum resolution of an optical system to be about 200 nm in the X-Y (lateral) direction. (In addition, a theoretical limit of about 500 to 700 nm exists in Z [depth].) This means that many subcellular structures and dynamics are simply not large enough to be observed in sufficient detail using most optical microscopes.
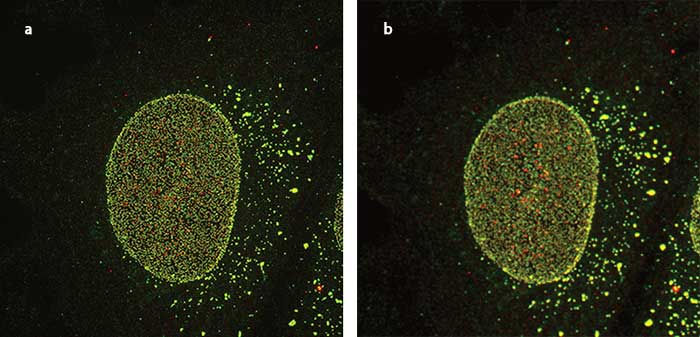
Nuclear pore imaged using supperresolution (a) and confocal (b) imaging. Courtesy of Ei Kosako Mikoto, University of Tokushima, Setsuro Fujii Memorial Center.
This 3-D representation of a cell nucleus reveals thousands of nuclear pores that mediate communication and exchange between the cell nucleus and the cytoplasm. The video was created from a series of live cell superresolution images to reveal details of the living system. Courtesy Ei Kosako Mikoto, University of Tokushima, Setsuro Fujii Memorial Center.
In the last 15 years, scientists have begun using superresolution microscopy techniques to image living phenomena beyond Abbe’s theoretical limit. In a relatively short time, these techniques and instruments have become popular in the study of far-ranging biological phenomena. Superresolution can be an ideal solution for biologists who need to resolve structures beyond the diffraction limit of optical microscopy, including those whose specimens are incompatible with electron or atomic force microscopy. This incompatibility may result from researchers’ need to study living samples in real time or because they require molecule-specific labeling achievable only through multichannel fluorescence microscopy.
The greatest advantage of superresolution as a method is its enhanced resolving power. There are numerous options for researchers who need to image samples at resolutions below 200 nm. Some techniques are reported to yield an improvement in resolution that can range up to an order of magnitude, allowing the observation of details on a cellular or molecular level that previously were entirely invisible. Each superresolution technique has its own capacities, advantages and disadvantages. Different superresolution techniques include:
Superresolution (a) and confocal (b) images of a growth cone fixed sample showing F-actin (green) and microtubules (red). Superresolution image captured using Olympus SD-OSR superresolution system. Courtesy of Kaoru Kato, Japan National Institute of Advanced Industrial Science and Technology, Neuroscience Research.
Evanescent wave. Eliminating background fluorescence increases the signal-to-noise (SNR) ratio and improves contrast and axial spatial resolution. If features of interest are located within 100 nm of the coverslip or tissue culture container, total internal reflectance fluorescence (TIRF) and related evanescent wave methods can enhance resolution. These methods take advantage of a phenomenon that occurs when light entering a system at a critical angle reflects at the interface between the coverslip and the sample medium. Reflection at this point, where higher and lower refractive-index media meet, creates a rapidly diminishing evanescent field within the sample medium adjacent to the interface. Because of the steep decline in excitation beyond 100 nm from the coverslip, unwanted background fluorescence and out-of-focus fluorescence are eliminated. TIRF microscopy provides excellent high-contrast imaging of single-molecule fluorescence and membrane dynamics.
Stochastic/localization techniques (PALM, STORM). Some superresolution techniques rely on the calculated localization of fluorescent molecules in the sample. Stochastic optical reconstruction microscopy (STORM) and photoactivated localization microscopy (PALM) switch continuously between fluorescent and dark states using specific fluorescent probes. By calculating the center positions of rapidly changing fluorescent molecules, the positions of the fluorochromes can be determined with high precision. Each image depicts random subsets of the fluorochromes within a specimen. A localization superresolution image is reconstructed from the position data accumulated over hundreds or thousands of individual images.
Localization methods such as PALM and STORM offer high spatial resolution (20 to 50 nm), but have low temporal resolutions ranging from 30 seconds to 30 minutes, depending on the application, sample and fluorochromes used. Recent advances in camera technology such as nondestructive readout offer the potential for even higher SNR ratios for single-molecule imaging using these techniques.
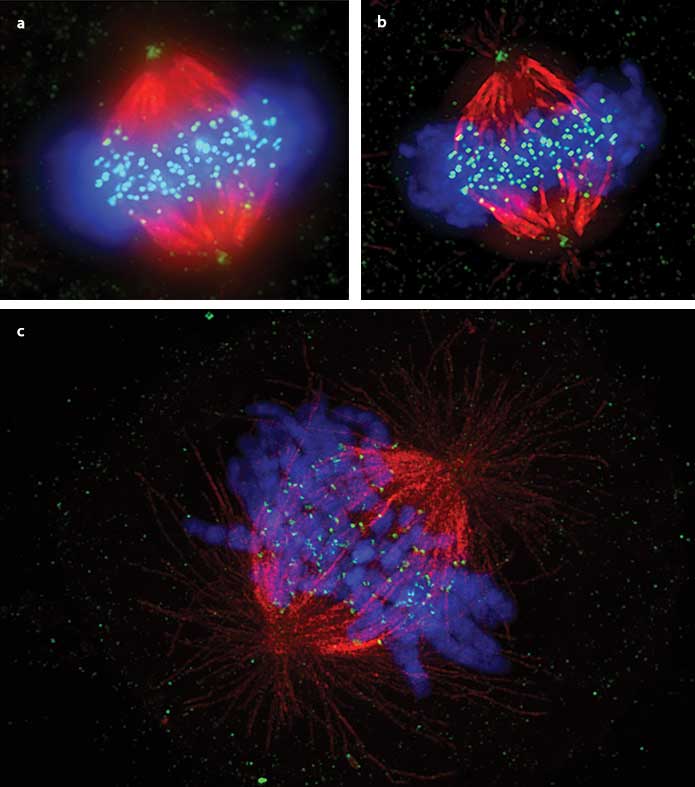
Visualization of somatic cell mitotic metaphase of the mitotic spindle, comparing widefield (a), deconvolution only (b) and superresolution (c).
Human cervical carcinoma-derived cell line HeLa cells were stained with anti-Hec1 antibody (kinetochores: green) and anti-tubulin antibody (microtubules: red), and immunostained with DAPI (chromosomes: blue). Deconvolution offers improvement in resolution. However, the superresolution image is the only one in which the binding of chromosomes to microtubules via kinetochores is observable. Courtesy of Masanori Ikeda and Kozo Tanaka, Tohoku University, Institute of Development, Aging and Cancer.
Emission depletion (STED). Stimulated emission depletion (STED) microscopy is a powerful superresolution technique that uses two laser beams sequentially, first to stimulate and then cancel out light. The first high-intensity laser excites the sample and stimulates fluorescent emission. The second laser, which is applied in a doughnut shape at the initial point of excitation, depletes all fluorescence except for that occurring in a tiny, sub-resolution volume of the sample. The instrument scans the sample, successively collecting image data coming from the excited fluorochromes at each scanned location.
STED has complex optical alignment challenges that can make it impractical for some researchers. And since STED images a small fraction of the fluorochromes it excites, the excitation necessary to produce an image can be damaging to samples. In addition, collecting image data can be time-consuming when working with a large field of view. However, STED can provide excellent results and, for small fields of view, can do so at high speed.
Structured illumination (SIM and (S)SIM). Structured illumination microscopy (SIM) involves exciting a sample with closely spaced repeating bands of light that interact with the specimen structure to produce moiré patterns. Algorithmic processing of the moiré patterns results in higher-resolution images than would be achievable using traditional unstructured widefield or TIRF microscopy. SIM can be performed with point scanning and spinning disk-based microscopes, for higher frame rates in imaging the dynamics of living systems. Saturated SIM, known as (S)SIM, is a related method that introduces nonlinear fluorochrome responses to produce higher-order harmonics, further enhancing SIM’s potential. SIM can provide as much as twice the native resolution of a widefield microscope and, depending on the implementation, imaging operates at a relatively high frame rate. This makes the technology particularly useful for imaging rapid events within living samples. In most practical applications, lateral resolution can approach 110 to 150 nm, with temporal resolution as high as one-to-three frames per second. More complex 3D SIM techniques, which produce resolution improvements by modulating excitation patterns in three dimensions, can produce axial resolution between 100 to 200 nm; however, sample thickness is limited to about 10 microns.

Challenges persist
Despite its enormous potential, superresolution has its difficulties, and challenges tend to increase as resolution improves. As systems image smaller and smaller detail, for example, the population of responding fluorochromes decreases, necessitating the development of new fluorochromes with higher quantum yields. Vibration and spherical aberration are a greater hindrance at higher resolution than they are at lower resolutions. In addition, some living systems are more adversely affected under superresolution imaging than others because of high excitation intensity or long exposure times. Eventually, systems can reach a point of diminishing returns, where stress upon the specimen and the potential impact on data credibility can outweigh the benefits of increased resolution.
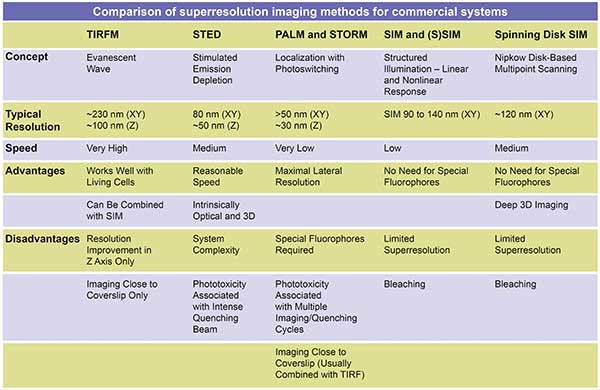
The quality of superresolution images also varies enormously. Published images that seemingly were captured using the same resolution vary dramatically in detail and appearance, and many have artifacts or errors. Lack of flexibility is another challenge for most superresolution systems. Many hardware-based systems have limited applications and require that specific fluorescent probes be used, so they might not work once an experimental protocol changes.
Finally, superresolution systems are expensive. A fully outfitted superresolution system starts at more than double the price of a confocal imaging system and may go upward of four times the price. The difference in cost compared to a widefield fluorescence system is even more dramatic.
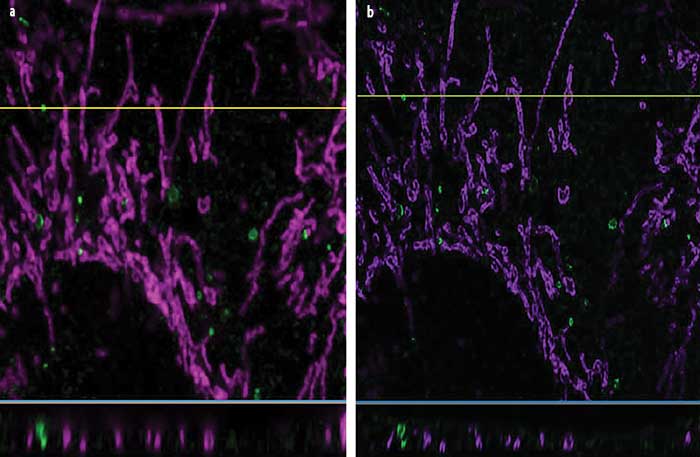
Comparison of autophagy captured using confocal imaging (a) and the Olympus OSR superresolution algorithm with 3D deconvolution (b), demonstrating enhanced resolution. The OSR approach produces lateral resolution improvement (top panels), and the application of deconvolution to the superresolution volume produces dramatic axial resolution improvement as well (lower panels). Courtesy of Ikuko Honda and Mizushima Noboru, University of Tokyo Graduate School of Medicine, Molecular Biology Department.
The best way to approach superresolution imaging is to start with the specific needs of a study and consider the validity of all approaches. This should include widefield and confocal imaging as well as superresolution techniques. The researcher should opt for whichever method produces the most usable fluorescence emission while providing the necessary data. With confocal microscopy, for instance, lowering the background intensity against which objects are imaged and localized improves fluorochrome visualization and image analysis. Sometimes, that enhanced contrast alone is dramatic enough to provide sufficient resolution of observed detail. In other cases, higher resolution is required.
Some systems allow researchers to opt for both traditional confocal and superresolution modes in a single instrument. Such systems allow the scientist to select the technique that provides the best results for a given experiment, and, even more important, to take advantage of the common stage. The researcher can use the same slide, switching back and forth between confocal and superresolution modes to produce two data sets for comparison. Multiple sources of information can lead to better certainty without artifacts. They also offer multiple perspectives to help confirm findings, much as researchers use electron microscopy to confirm optical microscopy findings in nonliving systems.
The Olympus SD-OSR microscope system is one such dual system. It pairs a high-speed spinning disk with high-performance optics and objectives that can deliver image resolution up to twice that of a widefield microscope. Resolution-limited excitation and higher SNR ratios combine with SIM image formation to deliver 110- to 130-nm resolution at up to three frames per second.
Another option for researchers is the application of deconvolution, a computational processing technique that incorporates a priori information about the system to improve contrast and resolution. Deconvolution is comparatively inexpensive and represents an intermediate step between widefield and superresolution. For some research challenges, it provides enough data to make the addition of specialized superresolution hardware, with all its challenges, unnecessary. In some cases, researchers can combine deconvolution with superresolution to enhance dynamic range and improve axial resolution.
With all its potential, superresolution has a way to go to reach its rightful place as a primary tool for advanced imaging. Use of consistent implementation and well-defined protocols is one way to help ensure that the data captured is consistent in the way it is collected and described from publication to publication.
Meet the author
Russell Ulbrich is manager of analysis and advanced imaging systems at Olympus Corporation of the Americas, Scientific Solutions Group, in Waltham, Mass.; email: [email protected].
Choosing the Right Tool
For researchers who want to select among the many diffraction-limited and superresolution tools, it is helpful to ask a series of questions to help find the best research tool for their application:
• What biological question do I want my experiment to answer?
• What deficiencies does my current imaging system have? What improvements would make a difference?
• Can I get what I need just by increasing contrast?
• Are there any issues in balancing specimen integrity against resolution gains?
• Can I achieve my goal with deconvolution?
• Is either system speed or flexibility important for future experiments?
• Is what I need to see so small that the research demands a stochastic or deterministic solution?
Every technique has strengths and weaknesses, so it is useful to consider all options before deciding which way to go.