Cavity ringdown spectroscopy promises to find atmospheric pollutants with ease and accuracy.
Sze M. Tan, Edward H. Wahl, Alexander Kachanov and Barbara Paldus, Picarro Inc.
What is air? Scientists worldwide continue to focus their attention and their instruments on answering that simple question with an ever-increasing standard for accuracy. It is no longer sufficient to know that air is composed mostly of nitrogen and oxygen. We need to know in detail about all the trace gases found in the air, the tens of parts per billion of ammonia, the several parts per million of methane and the 370 ppm of carbon dioxide. We must be able to identify and measure the hundreds of trace gas compounds that exist in the air we breathe, some at parts per trillion or even lower concentrations. By doing so, we can better protect ourselves from harmful airborne contaminants, and we can achieve a better understanding of the global climate and ecosystem.
A similar trend toward higher sensitivity and higher accuracy can be seen in instruments for the detection of trace contaminants in industrial gases. Gases play a vital role in industrial processes, such as semiconductor processing, oil refining, electric power generation, agriculture, glass manufacturing and waste treatment. As constraints on the processes used by all industries have tightened, forcing improvements to scrubbers and purifiers, the levels at which trace gases must be detected have decreased. Moreover, target gases are often found in complex background gas matrices comprising many other species, which can compound the difficulty of detecting them at trace levels.
Traditional optical gas detection
Historically, methods for trace gas detection have been based primarily on chemical reactions (electrochemical detection) or physical separations (gas chromatography). In response to the challenges of sensitivity and selectivity, a new technology based on optical absorption spectroscopy was developed. These optical detectors saw initial deployment in the 1980s and became widely used in the 1990s. Their basic principle of operation is simply to measure the difference in intensity between incident light, I0, and light transmitted through a path length, x, of sample, I(x,λ). Beer’s law relates the transmitted light at wavelength, λ, to the sample absorption, α(λ).

The absorption is related to the molecular concentration, C, of the target sample through the extinction coefficient, ε(λ), namely α(λ) = Cε(λ). The extinction coefficient can be measured from the slope of a calibration curve relating the concentration to the absorption. For any gas species, this curve is obtained by measuring the absorption for a series of different reference concentrations.
The absorption of a sample is determined by measuring the incident and transmitted light through the sample chamber and solving equation (1):
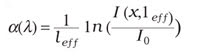
where leff is the effective path length through the sample, which may be greater than the length of the physical sample chamber if the light path is folded multiple times inside the chamber. From equation (2), it is clear that the absorption measurement is relative; i.e., it depends on the intensity of the incident light. It cannot distinguish between a true absorption change and noise incurred on the transmitted or incident intensities. Moreover, it directly depends on the effective path length of the measurement. This is a key limitation of traditional absorption spectroscopy and explains why these techniques require frequent calibration.
Equation (2) also shows that the ability of a spectrometer to detect a specific concentration depends not only on the light path length through the sample, but also on the intensity noise of the light source and detector. The minimum detectable absorption loss (MDAL) is found by normalizing the standard deviation of the instrument noise by the incident intensity and dividing the result by the effective optical path length. It typically is expressed in units of cm–1, and refers to the smallest detectable absorption change in 1 cm of path length during a single measurement. If many such measurements can be made within a short period, averaging may be used to reduce further (by the square root of the number of measurements) the threshold of detection achievable within that period. The sensitivity is defined as the achievable MDAL divided by the square root of the data acquisition rate in units of cm–1Hz–1/2. Sensitivity is a figure of merit for any absorption-based technique.
Typically, the concentration of the target species is obtained by measuring absorption peaks within its spectrum. Although many different species may absorb light at one or more similar wavelengths, the total spectral profile of any particular species is unique. The ability of a spectrometer to distinguish between two species absorbing at similar wavelengths is called selectivity. Because spectral features narrow as the sample pressure is reduced, selectivity can be improved by reducing the operating pressure. However, the instrument must still be able to resolve the resulting spectral lines. Thus, selectivity depends on spectral resolution. Spectral resolution, which typically is measured in frequency (megahertz), wavelength (picometers) or wave numbers (cm–1), is another figure of merit for a spectrometer.
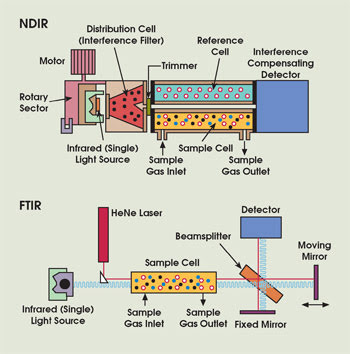
Figure 1. Both NDIR and FTIR spectroscopy use incoherent light sources. The light passes through the sample cell, and absorption at the spectral feature of interest is detected. The desired spectral region is isolated from the broadband light source emission either by using a filter (NDIR) or by dispersing the light and analyzing a specific section of the resulting spectrum (FTIR).
Common optical spectrometers such as nondispersive infrared (NDIR) and Fourier transform infrared (FTIR) use incoherent thermal light sources (Figure 1). The broadband emission of the light source passes through the sample and is either filtered (NDIR) or dispersed (FTIR) to measure only the absorption directly attributable to the target species. For both techniques, the physical length of the sample chamber limits their sensitivity. Some devices try to fold the light path several times through the chamber to improve sensitivity, but this approach encounters physical size and mechanical stability limitations. Typical FTIR cells have optical path lengths of several meters, and the longest reported cells have path lengths of several hundred meters. Typical FTIR and NDIR optical noise figures range from 0.1 to 1 percent, and MDAL for both range from 10–7 to 10–5 cm–1. These detectors, therefore, rely on measuring the strongest absorption transitions available, which are found in the mid-infrared (3 to 12 μm).
The spectral resolution of traditional spectrometers is also limited. NDIR methods use filters with relatively wide passbands, typically covering at least several rotational-vibrational lines, if not an entire rotational-vibrational band. The resolution of an FTIR spectrometer is limited by the distance over which it scans its interferometer mirror, and only large, high-resolution FTIR spectrometers can resolve individual spectral lines. Less expensive industrial FTIR spectrometers usually have resolution no greater than NDIR spectrometers, which are able to resolve only entire rotational-vibrational bands. Often, however, the strongest transitions will overlap with features of other species found in the sample mixture. The instrument designer must then make a sensitivity vs. selectivity trade-off.
Laser-based optical detection, often called tunable diode laser absorption spectroscopy (TDLAS), circumvents some of these problems by exploiting the coherent nature of laser light.1 A tunable, continuous-wave laser source brings two benefits: first, narrow linewidth, which allows high spectral resolution scans to be performed, and second, low spatial beam divergence and long temporal coherence length, which permits the beam to be folded on itself hundreds, if not thousands of times.
By transmitting laser light with a small beam size over long distances, multipass cells can be designed to achieve up to a kilometer of path length enhancement. Multipass laser spectrometers have demonstrated MDAL down to 10–9 cm–1, but they still remain limited by laser intensity fluctuations and interference fringes. As with NDIR and FTIR methods, standard multipass techniques rely on measuring the ratio of the absorbed to the incident light to determine the sample absorption. Because all of these methods are based on traditional absorption spectroscopy, they do not provide an absolute absorption measurement.
Unlike NDIR and FTIR methods, however, TDLAS methods exploit narrow-linewidth laser sources that are usually narrower than a single rotational-vibrational absorption line for a gas species at atmospheric pressure. Thus, TDLAS methods can measure isolated absorption peaks for a target gas and achieve excellent selectivity, compared with NDIR and FTIR.
Cavity ringdown spectroscopy
Cavity ringdown spectroscopy is a more recently developed TDLAS approach that replaces a multipass cell with a stable optical cavity. This technique benefits from the high spectral resolution of the TDLAS and multipass methods; however, unlike multipass techniques, ringdown spectroscopy is based on the principle of measuring the rate of decay of light intensity inside the cavity.2 This approach circumvents the traditional limitations of absorption spectroscopy and permits a direct and absolute measurement of the absorption.
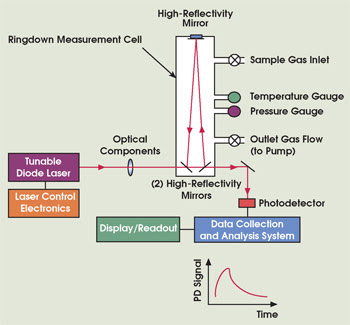
Figure 2. In cavity ringdown spectroscopy, light is injected into a high-finesse optical cavity formed by two or more mirrors. When sufficient light builds up inside the cavity, the light source shuts off, and a detector monitors the light transmitted through one of the mirrors.
Once sufficient light is injected into the cavity from a laser source, the input light is interrupted, and the decay of the stored light is transmitted out of the cavity through one of its mirrors and monitored using a photodetector (Figure 2). The transmitted light, I(t,λ), from the cavity is given by:
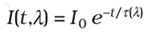
where I0 is the transmitted light at the time the light source is shut off and τ(λ) is the ringdown time constant. The transmitted light intensity decays exponentially in time.
For a given wavelength, λ, the decay rate, R(λ) = 1/τ(λ), is proportional to the total optical losses inside the cavity and equal to the empty-cavity decay rate plus a factor dependent on the sample absorption:

where R(λ,0) = 1/τ0(λ) is the empty cavity decay rate. The effective path length of the measurement is leff = cτ0(λ), where c is the speed of light. For typical mirrors having a reflectivity of 99.995 percent and scattering losses of less than 0.0005 percent, leff can be more than 10 km, and the path length enhancement can exceed 20,000.
Equation (5) shows that the sample absorption, α(λ) = ε(λ)C, can be found from the spectrum obtained by taking the difference between the decay rates of an empty cavity (C = 0) and a cavity containing a sample:

If the absorption cross section and line-shape parameters of the sample are known, then the concentration of the sample can be readily computed. (Detailed mathematical treatments of ringdown spectroscopy can be found in reference 2.) In contrast to traditional absorption spectroscopy, equation (5) demonstrates that a ringdown spectroscopy measurement is dependent neither on the initial intensity of the light inside the cavity, nor on the physical sample path length, provided that the signal has a sufficient signal-to-noise ratio at the detector.
Thus, ringdown spectroscopy is an absolute absorption measurement technique, immune to many of the types of drift that plague NDIR, FTIR and multipass TDLAS. From these absolute measurements, a robust, highly accurate, self-referencing concentration measurement can be obtained. Once the spectral parameters for a specific absorption feature are known, it can provide highly accurate concentration measurements without requiring frequent recalibration.
The MDAL for a ringdown spectroscopy system can be computed from equation (6):

As with traditional absorption methods, its MDAL is inversely proportional to the effective optical path length. However, because ringdown spectroscopy measures the rate of decay (or time constant) of the exponential ringdown waveform after the light source is decoupled from the cavity, it is insensitive to changes in the intensity of the light source. Therefore, unlike traditional absorption spectroscopy, its MDAL is independent of light intensity noise but rather depends on the instrument’s ability to measure the rate of decay, or ringdown time constant. This ability is called the “shot-to-shot” noise of the system and is written as Δτ/τ. In the equations for MDAL, the shot-to-shot noise term in equation (6) replaces the intensity noise term in equation (2). For a 20-cm-long sample cell, the effective path length is 8 km. A typical ringdown spectroscopy system can achieve a shot-to-shot variation of 0.05 percent and can easily achieve an MDAL of 5 × 10–10 cm–1.
Ringdown spectroscopy routinely achieves higher sensitivities than multipass TDLAS, by a factor of at least 10, based on effective path length alone. To date, the highest sensitivity achieved is 1 × 10–12 cm–1Hz–1/2.3 Moreover, a stable optical cavity can accomplish this sensitivity enhancement for sample volumes as small as 25 ml, compared with traditional multipass cells, which require volumes of 1000 ml. The benefits of smaller sample volumes manifest themselves in faster flow rates through the system and reduced requirements on the amount of sample used. Smaller volumes also allow less opportunity for samples to adhere to the sample chamber so that previous samples can be purged adequately. Thus, the ringdown spectroscopy system reduces system “memory,” which means that a sample measurement outcome is not affected by the sample concentration history.
New spectral horizons
The commercialization of TDLAS methods, which rely on the affordability and reliability of telecommunications lasers such as distributed feedback semiconductor diode lasers, has been constrained by the availability of such lasers at different emission wavelengths. The telecommunications sector has focused on several regions in the near-infrared: Short-haul transmitters operate at around 1310 nm, while long-haul multiplexed systems exploit the gain of erbium-doped fiber amplifiers in the 1510- to 1630-nm range. In some sense, the availability of telecommunications lasers emitting at species-specific wavelengths had gated the availability of sensors for those species. Nonetheless, TDLAS has been used to measure a long list of target molecules: carbon monoxide, carbon dioxide, methane, nitrous oxide, hydrogen cyanide, ammonia, hydrogen sulfide, acetylene ethylene, hydrogen fluoride, water, arsine and silane.
Efforts are under way to extend TDLAS to mid-infrared wavelengths where even higher sensitivities can be achieved by exploiting the stronger absorption features of fundamental transitions. Mid-infrared lasers with properties similar to distributed feedback, such as quantum cascade lasers, are allowing scientists to measure species whose near-infrared overtone transitions are simply too weak to see, even with ringdown spectroscopy. For example, excellent sensitivities were obtained for nitric oxide (NO).4
Furthermore, researchers are developing novel, broadly tunable mid-infrared lasers to leverage the mid-infrared “fingerprint” region, where each gas species has a unique spectral signature. Laser systems based on optical parametric oscillators have been developed in both the 3-μm5 and 6- to 8-μm regions6 specifically for ringdown spectroscopy. By exploiting these laser sources, the MDAL can be leveraged to detect compounds at parts-per-trillion levels. The capabilities of ringdown spectroscopy also are being extended beyond measurements of gases to thin films, liquids and aerosols.
Industrial applications
TDLAS instruments, in general, have already been deployed in demanding industrial applications, often residing outdoors or monitoring the output of high-temperature combustion processes. TDLAS has been used to detect gases such as CH4 or hydrogen fluoride (HF) in a plethora of applications:
• Environmental applications of CH4 detection include emissions monitoring at greenhouses and landfills. CH4 is a controlled, trace impurity in semiconductor process gases such as nitrogen, oxygen, argon and helium. In the production and transportation of natural gas, it also is measured at compressor stations during gas processing for safety and loss prevention.
• Safety and emission control applications for HF include detection in pot rooms, scrubber vents and stacks during aluminum smelting, in alkylation units in oil refining and in the manufacture of fluorocarbons, and monitoring in nuclear fuel reprocessing. It also is detected as an environmental emissions hazard from waste treatment incinerators and semiconductor facility stacks.
Existing detectors are rugged but can detect only parts-per-million levels of these gases.
Current commercially available ringdown spectrometers can measure moisture (H2O), HF and CH4. Applications for these devices have been driven primarily by the gas purity requirements of the semiconductor industry, although they are slowly expanding to cover the other applications mentioned above. The environments in which these instruments are deployed — semiconductor manufacturing facilities or gas analysis laboratories — are relatively benign.
Recently, the purity requirements of gases present in next-generation semiconductor lithography equipment have spurred the need to develop high-sensitivity sensors capable of detecting 1 ppb or less of ammonia (NH3) in clean, dry air. This opportunity allows high-sensitivity optical techniques like ringdown spectroscopy to compete directly with mass-spectrometry-based instruments, such as ion-mobility spectrometers. An ion-mobility spectrometer ionizes the sample to give it an electric charge, accelerates the resulting ions employing an electric field, and measures both the transit time of the ions through a chamber of known length and the electric current generated when the ions hit the end detector. The ionic current is calibrated to known concentrations; i.e., the concentration measurement is relative, not absolute. The selectivity is determined from the transit time of a given molecule and is a function of the molecule’s mass and charge.
The ability to detect trace levels (ppb) of NH3 can be applied to other industrial processes, such as monitoring NH3 generated during NOx reduction processes in electric power generation or emissions from agricultural operations. The ability to detect NH3 in background matrices such as ethylene could find process control applications in petrochemical production or fence-line monitoring for emissions in fertilizer and chemical operations.
At Picarro Inc. in Sunnyvale, Calif., ringdown spectroscopy has been implemented using a triangular ring cavity (US Patent No. 5,912,740) and directly modulated diode laser sources (US Patent No. 6,466,322) (Figure 2). A ringdown spectrometer was developed specifically to measure the concentration of trace amounts of NH3 in a background matrix of air. The spectral lines of NH3 were fully characterized with the technique, and a pair of well-isolated near-infrared absorption transitions were selected for detection (Figure 3a). The absorption coefficients were studied as a function of temperature and pressure and were programmed into the ringdown spectrometer.
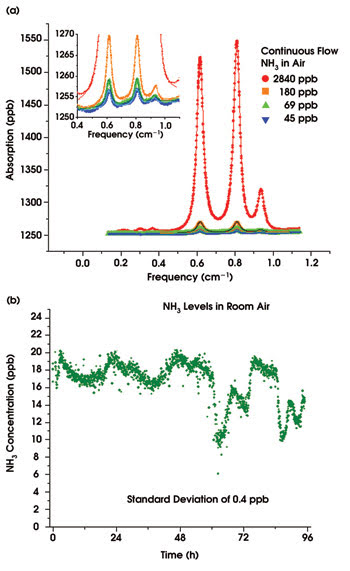
Figure 3. The near-IR spectrum of ammonia lines (a) shows three characteristic absorption peaks without interference from other species in the air. The graph recording the spectrometer’s dynamic range (b) illustrates the variations in concentration of ammonia in the lab air over several days. The detection limit is about 1 ppb.
In the lab
In a laboratory environment, these spectrometers have been able to achieve a routine sensitivity of 3 × 10–10 cm–1Hz–1/2 at a single wavelength. One can translate sensitivity into the lower detection limit for a substance, which is defined as the equivalent concentration that corresponds to three times the sensitivity. For the case of ammonia, the lower detection limit is 1.2 ppb for one second of data averaging. For industrial field applications, the lower detection limit is typically used as the figure of merit, while for spectroscopy research, sensitivity and MDAL are used as benchmarks when comparing techniques.
The NH3 lower detection limit for ringdown spectroscopy is comparable to that of ion-mobility spectroscopy. By exploiting its high spectral resolution, a lower detection limit can achieve excellent selectivity. Interfering species such as carbon dioxide, moisture, methane, acetone and isopropanol that are routinely found in the air of semiconductor cleanrooms do not confuse the spectral measurement because they are transparent in the NH3 detection region. Thus, the concentration of NH3 in air can be continuously monitored with high confidence that only the target gas is being measured, without the need for frequent calibration (Figure 3b). Unlike the ion-mobility technique, ringdown spectroscopy makes a direct measurement of NH3 concentration without requiring sample ionization.
Experiments with carbon dioxide (CO2) have shown that performance at the lower detection limit is superior to that of other optical approaches, such as NDIR, FTIR and TDLAS, in precision, accuracy, linearity, memory and zero drift. High precision and accurate measurements of CO2 are critical for environmental research on greenhouse gas emissions. For example, a zero drift of 0.01 ppm/°C was achieved, compared with 0.3 ppm/°C for the best NDIR instruments. A precision of one part in 2700 was achieved over a 15 °C operating range. Thus, ringdown spectroscopy is starting to demonstrate its viability as a very sensitive detection method for applications beyond the well-controlled semiconductor facility environment.
Ringdown spectroscopy has set foot on the road to commercial development and deployment that other optical absorption techniques, like NDIR and FTIR, already have traveled, but many hurdles remain. Reliability, customer qualification, volume manufacturing and cost reduction are but a few of the challenges that this technology will face as it competes with incumbent technologies and with those still to come. Yet, its potential benefits to numerous industrial applications will lessen the burden of bringing it to market. If NDIR and FTIR are like magnifying glasses in the trace gas field, it is our hope that ringdown spectroscopy will enable a new tool: the spectroscopic microscope.
Acknowledgments
The authors would like to thank members of Picarro’s marketing team, specifically Phill Amaya and Tom Steele, as well as from the R&D team, Bruce A. Richman, Chris Rella and Eric Crosson, for their help in writing this article. They also would like to thank Richard Zare and Marc Levenson for their support and guidance over the past two years.
References
1. W. Demtroder (1996). Laser Spectroscopy, Springer Verlag, Berlin.
2. K.W. Busch and M.A. Busch (1997). Cavity ring-down spectroscopy: An ultratrace absorption measurement technique. ACS Symposium Series 720, Oxford.
3. T.G. Spence, C.C. Harb, B.A. Paldus, R.N. Zare, B. Willke and R.L. Byer (2000). REV. SCI. INSTRUM., Vol. 71, p. 347.
4. A.A. Kosterev, F.K. Tittel, C. Gmachl, F. Capasso, D.L. Sivco, J.N. Baillargeon, A.L. Hutchinson and A.Y. Cho (2001). APPL. OPT., Vol. 40, p. 5522.
5. F. Kuhnemann, F. Muller, G. von Basum, D. Halmer, A. Popp, S. Schiller, P. Hering and M. Murtz (2004). Proc. SPIE, 5337, paper 18.
6. M.W. Todd, R.A. Provencal, T.G. Owano, B.A. Paldus, A. Kachanov, K. Vodopyanov, M. Hunter, S.L. Coy, J.I. Steinfeld and J.T. Arnold (2002). APPL. PHYS., B 75, p. 367.
Meet the authors
Sze M. Tan and Alexander Kachanov are research fellows at Picarro Inc. in Sunnyvale, Calif.
Edward H. Wahl is responsible for testing and qualifying cavity ringdown spectroscopy systems at the company.
Barbara Paldus is Picarro’s CTO and founder.