Transistor lasers are potentially much faster than diode lasers — and that’s just for starters.
Milton Feng, Nick Holonyak Jr. and Gabriel Walter, University of Illinois at Urbana-Champaign
Since it was first demonstrated by John Bardeen and Walter H. Brattain in 1947, the transistor has spawned a $300 billion semiconductor industry and has made possible our digital way of life, enabling computers, cell phones, MP3 players and the Internet. Now it is poised to give us even more. As a source of coherent light, the transistor laser offers the potential for much faster broadband communications, both for long-haul telecommunications networks and for short-haul connections within and between chips.
Moreover, there are bound to be applications that we cannot even imagine. For example, when the first practical visible LED was invented in the early 1960s, no one could have guessed that it would wind up in traffic lights and key chain fobs and that it eventually could revolutionize traditional lighting, becoming the basis of a global optoelectronics industry worth billions of dollars.
The light-emitting transistor and the transistor laser could have equally profound technological and economic impacts.
Over the past decades, the number of transistors on an integrated circuit chip has doubled almost every 18 months — so predictably, in fact, that the effect was dubbed Moore’s law. However, the physical size of the chip has remained relatively unchanged because of the limitations of metal interconnects. Metals are too resistive, and they slow the operation of microprocessors. If we were to make larger chips, the transistors would have to interact over greater distances. This would result in more-resistive paths and longer delays, limiting the speed and increasing the crystalline real estate that requires cooling.
To overcome these problems, chip manufacturers have been working to shrink the size and increase the density of transistors. Recently, they have sought to replace aluminum metallization with copper, which is a better conductor. If metals were replaced with superconductors, the chip size could be much larger and the processing power likewise would grow, but we have not yet been so lucky.
An alternative is the replacement of the metal electrical interconnects with optical ones. This poses its own problems, however, because the basic material of today’s integrated circuits — silicon — is not a good source of photons. Its indirect bandgap makes it a fundamentally poor light emitter, unlike the III-V materials used in today’s LEDs, lasers and high-speed heterojunction bipolar transistors. Nonetheless, there have been attempts to make silicon into a light emitter through various chemical and structural mutations, none of which has been particularly successful.
Researchers around the world have pursued the integration of optical and electronic components off and on since the early 1980s. Many efforts have centered on compound semiconductors, usually III-V crystals, for the obvious reasons: their high carrier mobility and good light emission. These materials offer so-called direct bandgaps, meaning that no momentum change is involved when an electron combines with a hole to create a photon.
As a result, compound semiconductors such as InP and GaAs, fabricated into PN junctions, are good light emitters when suitably biased, which makes it possible to construct the necessary light sources and detectors as well as transistors for integrated circuits from the same basic material. This is a huge advantage, potentially even overwhelming the advantages of silicon.
The III-V semiconductors offer all the ingredients for a diode laser or for a transistor laser. It took many decades to realize the latter device, but the transistor — bipolar by fact and by definition, operating with electrons and holes with carrier injection, recombination, transport and collection — always has been a light emitter. It just has not been a good one. It simply was not fully appreciated how important the base current is to transistor operation and how much room there is in the base, in spite of its thin size, to modify it with quantum-well layers and to change even further carrier recombination in a true transistor.
The transistor laser has revolutionary implications, both as a transistor and as a laser. As a transistor, it is easier to integrate on a chip than a diode because it has three, rather than two, terminals. Similar to integrated circuits, the transistor has a separate input and output, while the diode has the same input/output. As a laser, it promises speed advantages compared with a diode laser because its population inversion can be manipulated much more quickly with a drive current.
The energy gap defines and gives a semiconductor its special character, yielding a valence band more or less full of electrons below the band edge and a more or less empty conduction band above it. Electrons excited thermally (or with a photon) from the valence to the conduction band are free to conduct. The positively charged holes left in the valence band are equally free to conduct because of the adjacent electrons “bumping” from position to position.
Chemical doping of semiconductor layers with the appropriate atoms makes them hole-conducting P-type materials or electron-conducting N-type materials. This consequently enables the design and fabrication of relatively arbitrary layer arrangements in many forms, including, of course, PN junctions or heterojunctions (diodes) and NPN heterostructures (heterojunction bipolar transistors).
Consider a more or less standard PN heterostructure diode laser that has a quantum well in its central active waveguide region to improve its performance and that is forward-biased (N-side negative and P-side positive) so that electrons are injected at the top of the device and holes at the bottom (Figure 1a). The injected carriers congregate in the quantum well, where they have the lowest potential energy, and recombine across the bandgap to generate photons.
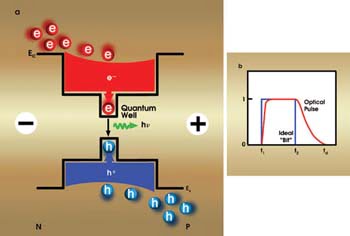
Figure 1. In this PN heterostructure diode laser under forward bias, injected carriers congregate in the quantum well, where they recombine across the bandgap to emit radiation (a). Ec and Ev are the conduction and valence band edge, respectively. At high drive frequencies, the optical response of the diode laser, indicated in red, does not follow the drive-current modulation, indicated in blue (b).
If the process is sufficiently rapid, stimulated emission is possible. Laser operation is autocatalytic: Electron-hole recombinations in the quantum well create radiation that stimulates the recombination process, which, in turn, strengthens the electron-hole coupling and recombination rate and narrows the linewidth of the emitted radiation. The resonator formed by the semiconductor “box” in which all this nice stuff is happening augments the process by bouncing the photons back and forth for repeated passes through the quantum well.
If the diode laser’s drive current is modulated, two factors affect the speed of the optical response: the speed of the recombination process and the rate at which photons escape from the cavity. In other words, if the drive current is turned off instantaneously, optical emission will end only after the initial population inversion has been depleted and all the photons have drained out of the resonator. As the modulation frequency increases, a point eventually is reached at which these factors limit the response, so an output optical pulse does not follow the electrical input pulse (Figure 1b).
Now consider a transistor laser. The transistor structure essentially is two diode structures, placed back-to-back. It is a three-terminal device, comprising the emitter, the base and the collector. (The emitter notation used for a transistor should not be confused with that applied to a diode light source. For a transistor, “emitter” refers to the terminal from which electrons or holes, rather than light, are emitted into the transistor structure.) Employing a similar injection process as the forward-biased diode, electrons are injected from the emitter into the base region of the transistor. The difference in potential energy across the heterojunction ensures that electrons are injected only from the emitter into the base, not vice versa.
The base region of the transistor is composed of highly doped semiconductor layers that provide a high concentration of built-in holes. Assuming that the base region is a direct-gap semiconductor, some of the electrons injected into this region will recombine with the built-in population and will emit light. This recombination process is identical to that of a diode laser.
The third component, the collector, is crucial to the speed advantage of the transistor laser. The collector is reverse-biased and, therefore, draws electrons out of the base region.
If the drive current to a transistor laser is instantaneously switched off, the population inversion is depleted more quickly than in a diode laser because the free carriers that do not rapidly recombine in the quantum well are swept out of the base. In effect, the collector reduces the carrier lifetime in the base by physically removing them, rather than by combining them with oppositely charged carriers. How quickly they are removed depends on the magnitude of the collector bias and on the thickness of the base region.
By reducing the dimensions of the base, therefore, the carrier lifetime can be made very small. The optical pulse still will lag the electrical signal because it takes a finite time for the photons to drain out of the resonator, but in practice, this time can be relatively short. In fact, base regions smaller than 40 nm have been implemented, resulting in transistors that operate at speeds greater than 700 GHz.
The injected carriers are captured directly or in multiple excursions over the quantum well, which governs the recombination and which delivers stimulated emission at high enough levels. By inserting an undoped quantum well in the P-type base (Figure 2a), the recombination and recombination radiation linewidth can be tailored, and the trade-off between electrical and optical signal strength can be set. The use of a quantum well offers as much as a fortyfold improvement in optical signal intensity, and the recombination and modulation speed can be taken in the direction of the transistor speed, toward hundreds of gigahertz.
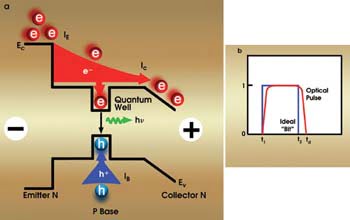
Figure 2. In the heterojunction bipolar transistor laser, the reverse-biased collector draws electrons out of the base region, reducing the carrierlifetime. Ec and Ev are the conduction and valence band edge, respectively, IE is the current out of the emitterregion, and IC is the current into the collector (a). The result is a much faster response than the diode laser, which enables transistor sources to operate at higher data transmission rates (b).
For data transmission, a built-in hole population and very short, transistor-determined electron lifetime provide potentially fast signal rise and fall times (Figure 2b). In principle, and in agreement with early data, transistor sources can operate at much higher data transmission rates than diode sources
The transistor laser is more than just a fast device, however. Recent work, although in its infancy, has revealed interesting potential applications. For example, the transistor laser can function as a high-speed nonlinear switch and signal mixer. Emitters with different wavelengths also are possible. The first light-emitting transistors and lasers featured infrared outputs, but they recently have generated red and blue light. Additionally, the mass production of transistor lasers is not far off, with the fabrication of 100 or more devices on a wafer already achievable.
The primary focus has not been on practical production, however. Rather, it has been on fundamental work such as what went into creating the point-contact transistor, the junction transistor, the silicon CMOS device, and LEDs and lasers — and resulted in the semiconductor microelectronics, display, lighting and optoelectronics industries. The light-emitting transistor and the transistor laser are at the beginning of what promises to be a new era in computing and communications.
The transistor, particularly the high-current-density NPN III-V semiconductor heterojunction bipolar device, now is an excellent light emitter. It offers stimulated emission, yielding a unique three-terminal laser and regime of transistor operation. In stimulated emission, the current gain shifts downward in magnitude as the transistor collector I-V characteristics compress and exhibit a detailed structure corresponding to the nuances in carrier recombination, mode turn-on and turn-off, and breakdown effects.
New opportunities are emerging for lasers, for transistors and for a natural active element for optoelectronic integrated circuits.
Acknowledgment
The authors are grateful to DARPA for support of this work under HR0011-04-1-0034 and to K.-Y. Cheng for insightful conversations.
Meet the authors
Milton Feng is the Nick Holonyak Jr. professor of electrical and computer engineering at the University of Illinois at Urbana-Champaign; e-mail: [email protected].
Nick Holonyak Jr. is the John Bardeen professor of electrical and computer engineering and physics at the University of Illinois and is the inventor of the visible semiconductor LED, the visible semiconductor laser and the quantum-well laser; e-mail: [email protected].
Gabriel Walter is a senior research scientist at the Micro and Nanotechnology Laboratory at the university; e-mail: [email protected].
Further reading
N. Holonyak Jr. (November/December 2000). From transistors to light emitters. IEEE J SEL TOP QUANT, pp. 1190-1200.
M. Feng et al (Sept. 26, 2005). Room temperature continuous wave operation of a heterojunction bipolar transistor laser. APPL PHYS LETT, 131103.
W. Hafez, W. Snodgrass and M. Feng (Dec. 19, 2005). 12.5 nm base pseudomorphic heterojunction bipolar transistors achieving fT = 710 GHz and fMAX = 340 GHz. APPL PHYS LETT, 252109.
N. Holonyak Jr. and M. Feng (February 2006). The transistor laser. IEEE SPECTRUM, pp. 50-55.
M. Feng et al (Feb. 6, 2006). Signal mixing in a multiple input transistor laser near threshold. APPL PHYS LETT, 063509.