Attosecond-pulsed photonic sources could introduce whole new fields of research, though brighter, more compact, and cost-effective sources must first be developed.
MARIE FREEBODY, CONTRIBUTING EDITOR
Attosecond photonics
allows for deep insights into material dynamics on ultrashort timescales and at nanometer resolutions. Because the attosecond is the natural timescale of electron motion within atoms, molecules, and solids, the applications for attosecond photonics range from research in physics, chemistry, medicine, the life sciences, and perhaps even manufacturing.
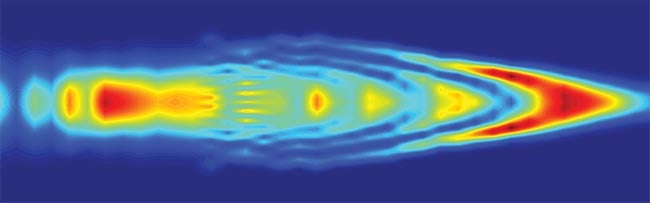
A measurement of the space-time structure of an attosecond pulse, as seen in the nonlinear medium where it is generated. Courtesy of University of Ottawa.
Although generating light in this domain is neither easy to accomplish nor to maintain, its eventual mastery could benefit a considerable number of markets, including petahertz-scale electronics, ultrafast metrology for microscopy or nanoscopy, and possibly the ability to control quantum processes.
Since the laser was first invented in the 1960s, pulse durations have shrunk from microseconds to femtoseconds (1 fs = 10−15 of a second). Then in 2001, an important milestone in ultrafast photonics was reached. For the first time, laser physicists were able to break the femtosecond barrier to create an attosecond pulse (1 attosecond = 10−18 of a second) — the shortest light pulse duration ever generated in the laboratory.
Attosecond science has largely remained within the confines of the laboratory in the last two decades, but technological advancements are under-way to develop high-repetition rate industrial-grade ultrafast and ultra-intense laser sources. Such advancements would finally springboard the attosecond field into the commercial domain.
Speeding up electronics
Electron dynamics incite most chemical reactions, including the absorption of light in a plant or a solar cell, and they are responsible for the switching in modern electronics.
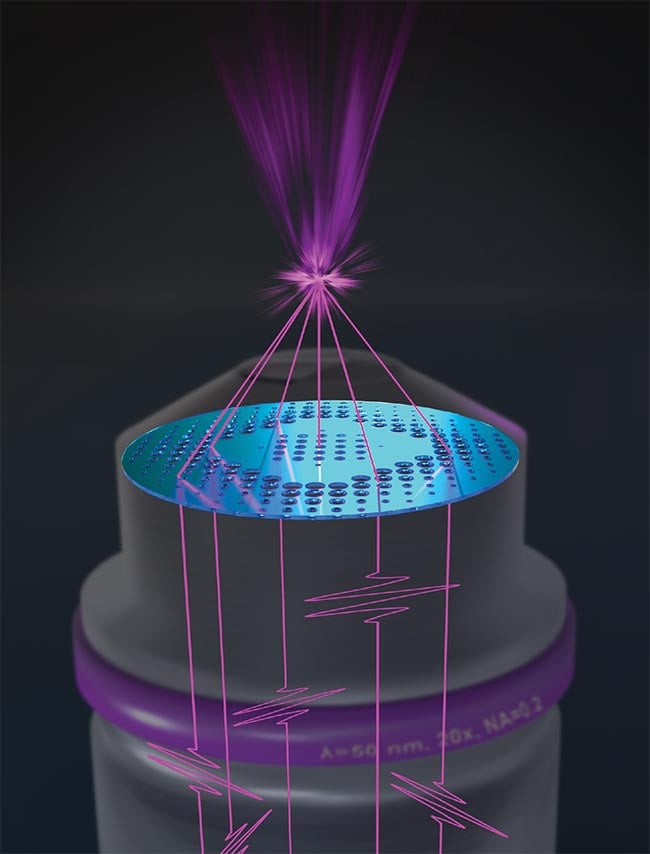
A depiction of a novel metalens focusing extreme ultraviolet (EUV) attosecond light pulses generated by high-harmonic radiation. Researchers believe such metalenses will transform attosecond spectroscopy into a microscopy technology. Courtesy of Harvard SEAS/Second Bay Studios.
Though observing electronic dynamics would be very useful, few techniques offer the required attosecond time resolution to do so.
“Understanding efficient light harvesting, building even faster electronics, and achieving room-temperature quantum communication will all require knowledge we can only obtain using attosecond pulses,” said Marcus Ossiander, a researcher at Graz University of Technology, Institute of Experimental Physics, Austria.
Conventional electronics face limitations due to heat accumulating within the processors. Because switching in a logic transistor only goes one way, switching it on requires it to return to its nonconductive state through energy dissipation before it can process further information.
“Ultrafast electronics with single-femtosecond or -attosecond optical gate signals could entirely waive the limitation
when switching between electronic states,” said Martin Schultze, professor of experimental physics at Graz University of Technology. “As long as the excitations remain coherent, intentional switching both ways becomes feasible.”
Schultze and his colleagues are already demonstrating solid-state electronic protocols that are around six orders of magnitude faster than current electronics.
They are also exploring pathways to dissipation-free operation.
Current experiments still struggle with stability and data-acquisition times. This could be solved with the development of a turnkey source that delivers single-cycle optical waveforms with microjoules of energy at a high repetition rate.
Today’s laser systems are working at repetition rates from 10 Hz up to a couple of kilohertz. However, to reduce imaging time and to be useful for most real-world applications, the repetition rate must be significantly higher, achieving hundreds of kilohertz and above.
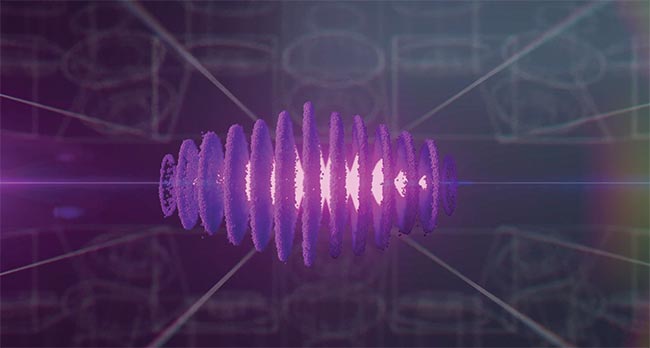
A 3D rendering depicting ultrafast coherent x-ray light in the undulator component of an x-ray free-electron laser (XFEL) that was seeded by a laser wakefield-generated electron bunch. Courtesy of TAU Systems.
Such sources are produced in the
extreme ultraviolet (EUV). But were they engineered at a central wavelength nearer to the visible range, they could be more easily handled with available optics.
“This would allow us to transfer the beauty of attosecond spectroscopy and sensing from quantum optics laboratories to applied and industrial research settings that require ease of operation and daily availability,” Schultze said.
From gases to solids
Attosecond pulses are typically generated using high-harmonic generation, which occurs when an intense infrared laser pulse is focused on a material to prompt strong nonlinear interactions. This process converts a tiny fraction of the pulse’s power to generate the high harmonics of the pulse’s optical frequency.
The first materials used to generate attosecond pulses were predominantly gases. This includes a jet of noble gas with a density of ~0.1 standard atmosphere (atm) over a length of ~1 mm. Or a cell filled with gas could be used, in which the number of atoms is <0.01 atm/cm.
High-harmonic generation is facilitated today through work performed by Donna Strickland and Gerard Mourou that earned them a Nobel Prize in 2018.
In their work, they used chirped-pulse amplification to stretch, amplify, and then compress ultrashort weak light pulses from a titanium-doped sapphire (Ti:sapphire) laser to produce high optical intensities.
Today, there is a strong shift away from Ti:sapphire sources in favor of fiber laser technology due to the latter’s better maintainability and cost. Both types of lasers, however, function in a similar manner. In fiber lasers, a fiber oscillator first generates weak light pulses, which are then amplified in a fiber-based chirped pulse amplifier.
When you have light pulses with electric fields strong enough to separate bound electrons from atoms (i.e., >1014 W/cm2 for gases, and >1013 W/cm2 for solids), you can then drive high-harmonic generation to obtain attosecond pulse trains, which are sequences of very short light pulses equally spaced in time,
Ossiander said.
In the process, a laser ionizes a material and accelerates the unbound electrons. Each electron releases this kinetic energy as a single high-energy photon when it eventually re-collides and recombines with its parent ion.
The process creates light pulses of
attosecond duration because the liberation of electrons can only happen at the very maximum of the light pulse’s oscillating electric field.
This also explains why the method usually generates a pulse train.
“There are usually many electric field maxima in a light pulse that can each create an attosecond pulse,” Ossiander said. “If you want to obtain a single attosecond pulse, you have to either use a very short light pulse to drive the process or use a light pulse with a complex polarization or color.”
But ever since the high-harmonic generation method was found to apply similarly in solids, researchers are increasingly moving away from simple noble gases to generate attosecond pulses using more complex samples such as liquid crystals, semiconductors, and other transparent solids.
In the case of solids, band-to-band transitions within the solid replace bound-to-continuum transitions in gases. The electron moves through the lattice of a solid instead of the free space around a gas atom before re-colliding.
This shift toward more complex samples helped pave the way for a future generation of integrated attosecond sources that use less power and do not need a constant supply of generation medium.
Moreover, it represented a step toward sources that are more compact and cheaper to operate.
Making movies in modern electronics
Mastering the attosecond time regime also enables new possibilities for molecular imaging. Soft x-ray beams can be made to focus to the diffraction limit by controlling the visible or infrared driving laser beam or through the use of metalenses.
“X-rays will allow imaging down to the wavelength limit through coherent diffractive imaging,” said Paul Corkum, professor at the University of Ottawa in Ontario, Canada. “Thus, we could combine 2D imaging with field sensing.”
Since applied voltages on a chip can
perturb harmonic generation, an applied voltage would effectively become
“visible.”
Today, Corkum focuses his work on metal samples in hopes of improving efficiency by using electrons that are already free from an atom. He is also exploring seeding x-ray laser transitions with the high harmonics of attosecond sources.
“The aim would be to combine the exquisite control over soft x-rays that is offered with attosecond technology with a gain medium to provide added energy,” Corkum said. The current limit in x-ray photon energy is 1.3 keV around the 4000th harmonic.
“I believe that it will be possible to take a movie of a functioning circuit,” Corkum said. “Ultrafast electronics combine small with fast. So does attosecond technology.”
To record a movie of the inner workings of electronic circuitry, spatial resolution must be added to the attosecond toolbox.
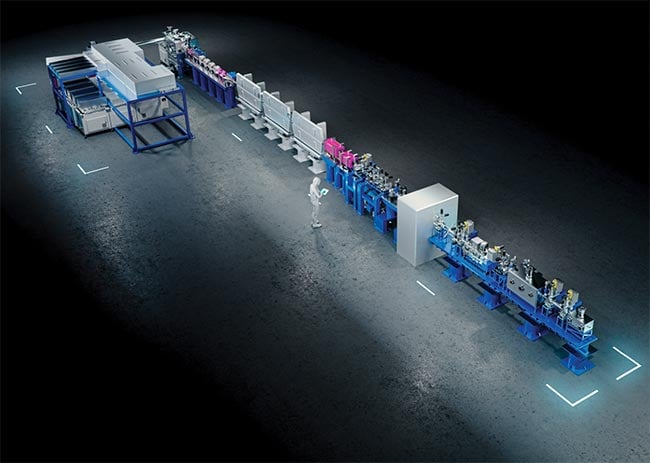
A 3D conceptual rendering depicting the envisioned TAU x-ray free-electron laser (XFEL) including one user end station. The image shows how compact XFEL machines could be in the near future. Courtesy of TAU Systems.
Because transparent materials do not exist in this range of frequencies, new optical concepts, such as metalenses, are being developed to help achieve such imaging capabilities.
Federico Capasso’s group at the Harvard School of Engineering and Applied Sciences demonstrated a metalens that has the potential of enabling high-resolution microscopy in the EUV region, claiming
it is the first transmissive lens in that spectral region.
“Until this work, focusing in the EUV could be achieved only using bulky and complex optics, such as mirrors and Fresnel zone plates,” Capasso said. “Metalenses are flat and thin, and optical aberrations can be corrected much more easily than in conventional refractive optics.”
Ionizing radiation with attoseconds
Attosecond-pulse generation using high-order harmonic generation achieves much higher photon energies through frequency up-conversion of the infrared source. Most attosecond pulses produced this way are in the EUV or soft x-ray range.
This ionizing radiation can be useful for studying biological molecules, such as the DNA building block adenine, and for investigating their response to stimuli.
This is exactly what Francesca Calegari is exploring in an attempt to control the dissociation of molecules by acting at extreme timescales. Extremely short light pulses act directly on an electron’s position or on the distribution of electrons within a molecule, which impacts bond formation and breaking.
“I strongly believe that attosecond technology has opened new and exciting possibilities to control chemical reactions by acting at the electron timescale,” said Calegari, head of the Attosecond Science division at the German Electron Synchrotron (DESY) in Hamburg, Germany. “Chemists tend to neglect the role of the electron dynamics, but with a proper sequence of ultrashort light pulses, we
are now able to control the ionization-induced dissociation of molecules by taking advantage of the electron density distribution before the nuclei even start to move.”
Chiral recognition is crucial in many fields, including drug engineering, enantio-selective catalysis, and biophysics.
Influencing the electron density distribution in a molecule has a direct bearing on a molecule’s reactivity. Harnessing this reactivity could improve biological functionalities, such as light harvesting.
For example, Calegari’s group is currently investigating how to control the response of chiral molecules by exploiting the electron dynamics induced by ultrashort laser pulses. Chiral recognition is crucial in many fields, including drug engineering, enantio-selective catalysis, and biophysics.
Chiral molecules exist in two enantiomeric forms. In other words, the two forms share the same chemical formula, but they are mirror images of each other and have extremely different chemical properties. Initiating coherent electron dynamics in a chiral molecule offers a method to control the chiral response and therefore the chemical reactivity of the molecule without changing enantiomer. This would open a new pathway toward a charge-directed reactivity.
EUV and x-ray radiation
Although Texas-based TAU Systems is not expressly producing attosecond-pulsed sources, the company aims to develop compact, high-intensity x-ray free-electron lasers (XFELs) that could provide a potential route to generating commercially applicable attosecond pulses.
“XFELs are the highest-brilliance x-ray sources today and also allow us to tune the wavelength/photon energy of the x-rays,” said Björn Manuel Hegelich, a physics professor at the University of Texas at Austin and the founder of TAU Systems. “Current systems require kilometer-scale accelerators, making them both large and expensive. Compact, laser-driven sources have the potential to change that.”
In addition to providing broader access to high-intensity, coherent EUV and
x-ray sources for scientific, industrial, and medical applications, more compact XFELs could also allow more cost-
effective and practical pulsed sources operating in the attosecond domain.
“We can generate EUV and x-ray pulses for the first time in industrial-grade machines readily usable in research labs, medical facilities, or production
floors,” said Catalin Neacsu, vice presi-
dent of business development at TAU Systems. “With hard x-rays, one can peer deep inside metal objects, such as the deep welds in reactor vessels or power plants, and life inspection of operating metal smelting operations, the nondestructive quality control of additive-manufacturing parts, like medical
implants or aircraft engine parts.”