Flow cytometers, ubiquitous in biomedical, clinical, engineering, and environmental laboratories, may soon benefit from advancements in silicon photomultipliers.
SLAWOMIR PIATEK AND EARL HERGERT, HAMAMATSU CORP.
Flow cytometry is a technique that can provide quantitative and qualitative information about biological cells and microparticles. The information is in the light that has interacted with the cells as they pass one after another and one at a time through a narrow region illuminated by one or more lasers. The machine that enables such studies is a flow cytometer, ubiquitous in biomedical, clinical, engineering, and environmental laboratories. Its design consists of fluidics, illumination optics, photodetection, data analysis, and, in some models, cell sorting (Figure 1).
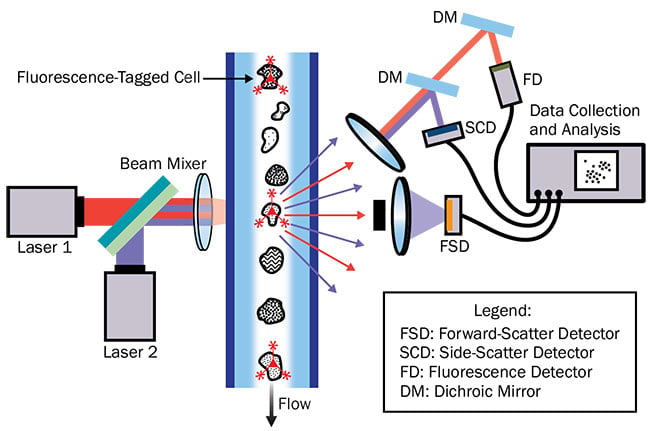
Figure 1. The basic layout of a flow cytometer. Courtesy of Hamamatsu Corp.
In the fluidics component, a pressurized and filtered sheath fluid flows through a flow cell — a narrow, round or rectangular tube whose diameter ranges between 50
and 250 μm. The flow is laminar. Upstream, before the laser interrogation point, a liquid — distinct from the sheath fluid, with suspended cells or particles — is injected into the central section of the flowing sheath. The liquids do not mix; instead, the faster-flowing sheath accelerates the sample liquid, creating a coaxial flow pattern: The inner flow is known as the sample core.
The hydrostatic pressure of the sample core is always higher than that of the sheath; the pressure differential controls the width of the sample core: The typical diameters range between 5 and 20 μm. The smaller the pressure differential, the narrower the sample core and the lower the flow rate of the core. Narrowing of the sample core causes the cells to flow near the central axis in a single-file formation. This hydrodynamic focusing is essential to ensure that only one cell at a time passes through the laser interrogation point (Figure 2).
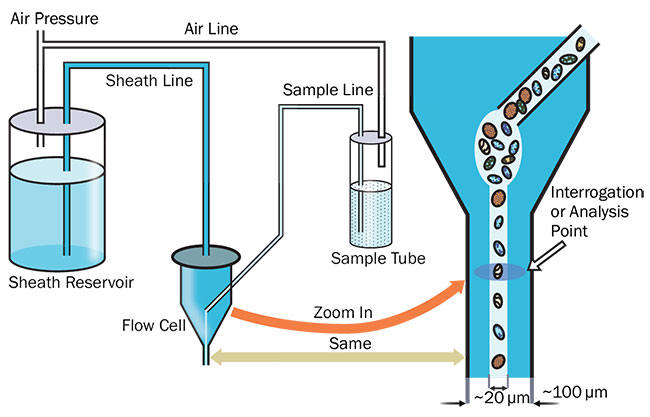
Figure 2. Fluidics and detail of the interrogation process. Courtesy of Hamamatsu Corp.
A typical flow cytometer uses at least
one laser for the illumination of the interrogation point, also known as the analysis point. The analysis point is actually a volume defined by the intersection of the sample core and the laser beam. In this volume, the laser light interacts with the passing cell and also induces fluorescence if the cell is tagged with a fluorescent molecule (known as fluorophore or fluorochrome).
The two requirements of flow cytometry
are: 1) only one cell at a time may be present at the analysis point, and 2) each
passing cell must be illuminated by the
same inity of light. Violation of the
first requirement can lead to cell misidentification because the outgoing light comes from multiple cells at the same time. Violation of the second will result in different quantities of outgoing light from identical cells. To improve the odds
of nonviolation, the profile of the focused laser beam at the analysis point is an
ellipse whose long axis (typically ~60 μm) is perpendicular to the direction of the flow. The short axis (typically ~20 μm) parallel to the flow represents the smallest permissible separation between the flowing cells.
A typical laser outputs a circular beam 1 to 2 mm in diameter with a Gaussian inity profile. The elliptical illumination is achieved by focusing the light with a cylindrical lens. The average separation between the cells is a function of the cell concentration in the core liquid (ranges from ~105 to 5 × 106 cells per ml) and the core diameter. The sampling rate of the
cells depends on this concentration and also on the speed of the core flow; a benchmark rate is about 1000 cells per second.
Stable light source
There are several considerations in selecting the appropriate light source. It should provide stable illumination at the
analysis point without damaging the
cells and should excite fluorophores if fluorescence tagging is employed. A laser fulfills these requirements well, though other sources, such as an arc lamp, can also
be used. Examples of some commonly used lasers are argon-ion, helium-neon, and red-diode. Their useful wavelengths are mostly in the 400- to 600-nm range.
At the analysis point, the cell can reflect, scatter, diffract, refract, and/or absorb the incident light. The cell can also fluoresce. The importance of these processes depends on the cell’s properties, such as size, shape,
index of refraction, granularity, and presence of either endogenous or tagged fluorophores. Some fraction of the outgoing light is incident on at least two lenses. The first, placed on the optical axis, collects the forward scatter signal, whose quantity is primarily determined by the cell’s size and index of refraction. The other lens, placed orthogonally to the optical axis, collects the side-scattered and fluorescence signals. The former is a function of the cell’s granularity, whereas the latter can be polychromatic and is typically of lower brilliance.
A combination of dichroic mirrors and long-pass and short-pass filters spatially segregates the side scatter and fluorescence light according to wavelength. Each monochromatic component illuminates the active area of a dedicated photodetector, which converts radiant energy into electrical energy. A flow cytometer may utilize some combination of three distinct types of photodetectors depending on the wavelength, inity, and pulse duration. These photodetectors are a photodiode (PD),
avalanche photodiode (APD), and a photomultiplier tube (PMT). A newly developed silicon photomultiplier (SiPM) — a photodetector whose sensitivity to
light can rival that of a PMT — may become the fourth choice.
Among the many optoelectronic characteristics of a photodetector, three are crucial in flow cytometry: responsivity,
dynamic range, and bandwidth. The first is a wavelength-dependent proportionality factor between the incident light power and the photodetector’s output current. The second is the range of incident light powers for which the photodetector outputs a linearly proportional current whose signal-
to-noise ratio (SNR) is >1. The third
can be thought of as the parameter that determines the likeness between the shapes of the output current pulse and of the incident light pulse.
Among the many optoelectronic characteristics of a photodetector, three are crucial in flow cytometry: responsivity, dynamic range, and bandwidth.
A photodetector is only one part of a complete electronic detection system. Each
aforementioned photodetector is essentially a light-driven current source. Either a resistor or current-to-voltage amplifier converts its output current pulse to a voltage pulse, which is then processed electronically to determine the amplitude, width, or area, depending on the design and research interests. The quality of these data points, as measured by SNR, depends not only on the optoelectronic characteristics of the photodetector but also on the noise characteristics of the front-end electronics. In addition, the front-end electronics together with the photodetector constrain the detection’s dynamic range and bandwidth.
Reading the light
The forward-scatter signal is typically high enough to warrant the use of a PD. It is a semiconductor device with a single PN junction. Most of the PDs in flow cytometry are made of silicon. They have excellent responsivity in the 350- to 1000-nm range, with the maximum of about 0.5 A/W around 900 nm. The peak output current depends on the power of the illuminating laser, properties of the cells, and the diameter of the objective lens. Its typical values are in the μA range, implying peak radiant power in the μW range.
Side-scatter and fluorescence signals
are weaker than the forward scatter signal. If a PD were to be used, one would expect the peak current on the order of hundreds of pA to tens of nA. Detecting such currents so that SNR >1 would require expensive low-noise front-end electronics. An alternative approach is to use a photodetector with an intrinsic gain, such as PMT, APD, or SiPM. In this approach, the intrinsic gain suppresses the importance of noise from the front-end electronics (Figure 3).

Figure 3. Importance of the photodetector’s intrinsic gain. Courtesy of Hamamatsu Corp.
A PMT is a cold vacuum tube whose main structural components are a light-sensitive photocathode, a sequence of
dynodes, and an anode (Figure 4). All of these components are electrically biased, with the photocathode at the lowest electrical potential and the anode at the highest (typically at the ground level). A photon incident on the photocathode causes a photoemission of electron into the vacuum. This electron can then initiate electron chain-
multiplication in the sequence of dynodes so that, effectively, a single photon can result in a million or so electrons collected at the anode (gain = number of electrons), yielding a typical anode responsivity of about 105 A/W in the visible range. Supply voltage (300 to 800 V) controls the gain and, thus, anode responsivity. The trade-off for having intrinsic gain is higher photodetector noise, commonly expressed as excess noise figure. Its typical value for a PMT is 1.2 to 1.4 (an ideal noiseless case is 1).

Figure 4. A photomultiplier tube (PMT) module. Courtesy of Hamamatsu Corp.
An APD is like a PD albeit with an intrinsic gain. The gain, with a maximum value of about 100, is due to impact ionization of silicon atoms in the depletion region. To achieve it, an APD is reverse-biased to a voltage close to but less than the breakdown voltage (150 to 400 V). With such bias, the APD is said to operate in the linear mode. APDs have rather large excess noise, with typical values around 3.5. Biasing an APD above the breakdown voltage makes the APD operate in Geiger mode. (Note that APDs are constructed to operate either in the linear mode or in the Geiger mode but not in both.) In this mode, an APD is effectively a light switch — on or off — and requires an external circuitry to recover from the off state.
SiPMs
Developed in the mid-1990s, a SiPM is a rectangular array of square microcells, all connected in parallel. Each microcell is a series combination of Geiger-mode silicon APD and a quenching resistor, whose role is to make the APD recover from Geiger discharge. SiPMs are small, two-pronged devices requiring a biasing voltage of only of volts. Depending on the model, the active area can be from
1 × 1 to 6 × 6 sq mm, consisting of several hundred to of thousands of microcells. The size of a microcell ranges from 10 × 10 to 100 × 100 sq μm. Dividing the active area of a SiPM by the area of its microcells yields the total number of microcells. This number and the dark count rate are the key parameters affecting the dynamic range of a SiPM.
A SiPM has a gain that is comparable to that of a PMT, and its excess noise is only about 1.1 to 1.2. Moreover, its responsivity and bandwidth resemble those of a PMT too. Examples of additional desirable characteristics are durability, immunity to a magnetic field, lack of warmup time, and simpler biasing and readout electronics. Given these advantageous characteristics, should we expect that the SiPM will completely replace the PMT in future generations of flow cytometers?
Predicting the future is difficult, so it may be easier to state why the replacement has not already happened. It is the smaller dynamic range of a SiPM that hampers the transition. Compared to a SiPM, a PMT has lower dark count rate per unit active area. The consequence is that a PMT can detect a fainter light signal than a SiPM. As the incident light level increases, both devices will eventually become nonlinear and, therefore, approach the upper limit
of their dynamic range. In the case of a PMT, this will occur when the peak anode current reaches the maximum rating. In the case of a SiPM, the finite number of microcells limits the dynamic range. As the number of photons in a pulse increases, it becomes progressively more likely that more than one photon will illuminate
a microcell at a given moment, and that this illumination could occur while the microcell is in the recovery stage.
The upper limit of the given SiPM’s dynamic range cannot be changed: It is constrained by the total number of microcells. However, the lower limit can be extended to fainter light levels by cooling
the device, which decreases the dark
count rate. Cooling the PMT does the same. The upper limit of the PMT’s dynamic range can be extended in two ways. One is to lower the gain by decreasing the supply voltage, and the other is to use a voltage
divider circuit that increases the maximum anode current rating. Neither of these approaches is viable for a SiPM.
Research and development of SiPMs is
ongoing, yielding an improved performance with every new generation of the device. As the dark count rates come down and the total number of microcells goes up, the odds that a SiPM becomes a part of a functional flow cytometer are excellent.
Meet the authors
Slawomir S. Piatek has been measuring proper motions of nearby galaxies using images obtained with the Hubble Space Telescope as a senior university lecturer of physics at New Jersey Institute of Technology; email: [email protected].
Earl Hergert is a vice president of marketing at Hamamatsu Corp. in Bridgewater, N.J. He has worked at Hamamatsu for 28 years; email: [email protected].