Advanced laser designs are putting a new complexion on the noninvasive diagnosis of skin disease by accelerating scan times, improving resolution, and providing more data.
KEITH OAKES, ELFORLIGHT LTD., AN AMS TECHNOLOGIES COMPANY
The earlier that skin diseases are detected, the greater the odds that
a particular therapy will be effective and successful. Optical
dermoscopy, today’s medical
standard for achieving early detection, allows experienced dermatologists to
reliably find superficial changes denoting potential disease in the skin. But the method is
not effective for early diagnosis of mutated structures in deeper layers of the skin. Until recently, such diagnoses required the histological evaluation of biopsies, which can cause pain and scars for the patient, and histology usually only provides results after days.

Skin tissue with a melanoma (dark area) and the surrounding structure of very fine blood vessels. Multispectral photoacoustic imaging technology is a noninvasive technique that offers great penetration depth and high resolution. The short-pulse laser sources that drive these systems contribute significantly improved image data that can provide more detailed diagnoses of tissue under examination. See Reference 1. Courtesy of Mathias Schwarz.
Ultrasound can produce an image of various structural densities below the skin’s surface.
But its resolution is not sufficient to show
microfine blood vessels in the upper layers of
the skin. The number and density of these blood vessels in the microenvironment of tumors such as melanomas is a measure of the progress of
the disease, and the oxygen saturation in these tiny, finely branched vessels is important for diagnosis and therapy prospects.
A more compelling alternative is multispectral photoacoustic imaging, which — compared to ultrasound — offers higher spatial and temporal resolution. Additionally, its deep penetration depth up to several millimeters (with lower
resolution even in the centimeter range) is
superior to other optical techniques. Multispectral photoacoustic imaging employs a short
laser pulse that penetrates the tissue, which
absorbs the light radiation and expands thermoplastically to generate a response in the form
of ultrasound waves. Unlike conventional ultrasound instruments that generate a signal that “echoes” off biological structures to be picked up by a detector, photoacoustic imaging causes the structures themselves to generate the ultrasound.
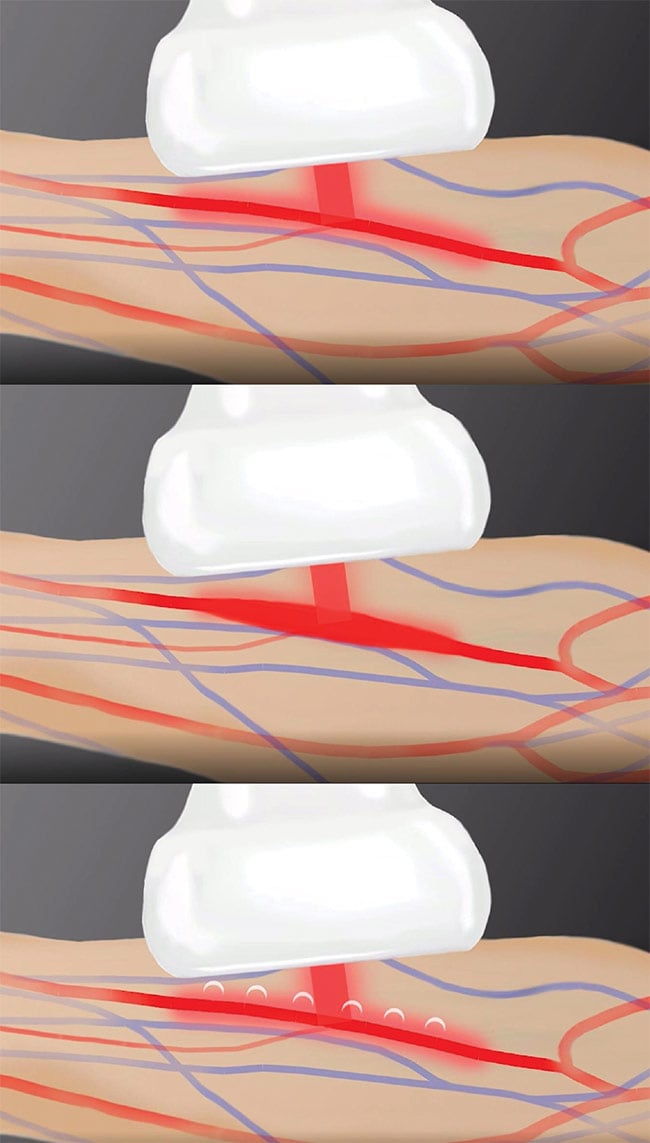
In photoacoustic imaging, the tissue is excited by means of a short laser pulse (top). The absorption of the laser light leads to the expansion of the tissue (middle), and the resulting emission of ultrasound pulses (bottom) are picked up by a detector that converts them into image information. Courtesy of iThera Medical.
This allows photoacoustic methods to not only identify blood microvessels but also to obtain functional and metabolic information. The intensity of the emitted
ultrasound signal depends on the wavelength of the laser, which, in turn, determines the optical absorption capacity of targeted chromophores in the examined tissue. Typical chromophores found in skin layers include melanin, oxygenated and deoxygenated hemoglobin, collagen, lipids, and water.
Tissue identification
To clearly distinguish biological components from each other, multispectral photoacoustic imaging relies on the fact that they each exhibit absorption maxima at different wavelengths. Deoxygenated
hemoglobin, for example, exhibits maximum absorption at 555 nm, whereas oxygenated hemoglobin shows a local absorption minimum. At 579 nm, this relationship is reversed, while at 606 nm the absorption of both types of hemoglobin is low and melanin dominates.

The absorption lines of typical skin and blood components. Absorption maxima with simultaneously reduced absorption of the other components occurs at 555 nm for deoxygenated hemoglobin (Hb), 579 nm for oxygenated hemoglobin (HbO2), and 606 nm for melanin. Courtesy of iThera Medical.
Current photoacoustic imaging systems that apply this multispectral approach, such as Munich-based iThera Medical’s RSOM system, gather absorption spectra data by scanning tissue successively using the aforementioned laser wavelengths. By using raster-scanning optoacoustic mesoscopy (RSOM), the system achieves details with resolution that falls between macroscopic and microscopic imaging. The RSOM technique sequentially scans the laser beam across the tissue surface to provide a larger examined area compared to fixed, single-point optics.
Until recently, photoacoustic imaging
was mainly considered a promising preclinical laboratory technique for users willing to assemble systems from individual components. With the commercial emergence of systems such as the RSOM, ready-made solutions can further include sophisticated software suitable for use in clinical research.
Fast scans avoid motion artifacts
Early multispectral photoacoustic
imaging systems relied on dye lasers
with a tuning range limited to about
30 nm and could switch between wavelengths only very slowly. Such systems could take minutes to complete a scan with one wavelength before switching to another wavelength to begin a second scan.
The technology was not suitable for
examinations on living organisms because movement artifacts can lead to inconsistent measurements, and it is difficult for patients to tolerate such long measurement times.

Until recently, photoacoustic imaging was largely a preclinical laboratory technique for users willing to assemble their systems from individual components. The commercial emergence of ready-made solutions has begun to change this situation and has further provided users with sophisticated software suitable for use in clinical research. Courtesy of iThera Medical.
Today’s RSOM systems overcome these slow scans by employing diode-pumped solid-state (DPSS) lasers that generate short pulses under 5 ns to deliver high energy up to 20 µJ. These lasers are able to emit at the key four wavelengths — 532, 555, 579, and 606 nm — in quickly interleaved laser pulses to achieve multispectral absorption measurements almost simultaneously at each individual pixel at a high frame rate. As a result, the systems considerably reduce or eliminate motion artifacts.
Raman shift adds more wavelengths
These RSOM systems rely on a 1064-nm, Q-switched, DPSS pump laser, the output of which is converted to 532 nm via a second-harmonic generator. The 532-nm laser beam is then directed onto a Raman shift unit consisting of a potassium gadolinium tungstate (KGW) crystal in an L-shaped cavity to precisely produce the Raman shifts (the Stokes wavelengths where key chromophores show specific absorption spectra).
The laser beam at the output of the Raman shift unit contains all four wavelengths, including the 532-nm fundamental, plus the first, second, and third Stokes wavelengths — respectively, 555, 579, and 606 nm. However, to clearly assign the ultrasound response, each wavelength must individually interact with the tissue in rapid succession. This requires a solution for separating and sequentially routing the wavelengths to the system’s output.
An acousto-optic tunable filter solves this task very elegantly. This filter consists of an optical crystal into which a transducer couples acoustic waves
that, in turn, deflect individual wavelengths of light passing through the crystal by a defined angle. The transducer of this filter is controlled by means of an electrical radio frequency signal, the frequency of which determines the wavelength of the specifically deflected light component.
Due to the underlying physics, the
various Raman wavelengths at the output of the shift unit show different pulse
energies. Here, too, the acousto-optic tunable filter provides a remedy: In addition to the wavelength to be deflected, an attenuation of the amplitude can also be selected for each of the wavelengths, so that all four wavelengths are present at the output with the same energy — as required for a correct measurement.
By cleverly routing the optical path inside the DPSS laser, only the light’s slightly deflected part reaches the laser’s output, where it is coupled into a fiber that guides it to the tissue to be scanned for examination.
Most systems for sequential, multispectral photoacoustic imaging use tunable lasers based on optical parametric oscillators for the sequential output of various wavelengths, which enables wavelength-switching frequencies in the region of only 100 to 200 Hz. Recently, laser developers at AMS Technologies’ subsidiary Elforlight achieved a switching frequency that reaches up to 2 kHz, about 10× faster and much more cost-efficient than optical parametric oscillator-based systems.
The energy of the emitted laser pulses should be high for good, high-resolution photoacoustic images. But the energy must not exceed specified limits to avoid tissue damage and patient injury. The shortest possible pulse duration is also crucial for the quality and resolution of the resulting ultrasound image. The Q-switched DPSS laser designs were proved to meet the necessary requirements with an optical pulse energy between 10 and 22 µJ and short pulse durations of less than 5 ns.
Short pulses for high resolution
The detection bandwidth and resolution of photoacoustic imaging is limited by the finite duration of the incident laser pulses. The boundary condition for ideal measurements based on the photoacoustic
effect is defined by so-called stress confinement, in which the stimulating laser pulse is much shorter than the typical time constant for a voxel’s acoustic relaxation. If, however, the pulse duration is within the range of this time constant, the finite pulse duration represents a low-
pass filter. The longer the pulse, the
lower the cutoff frequency of the low-pass filter.


Raster-scanning optoacoustic mesoscopy (RSOM) employs a photoacoustic imaging technique that scans a multispectral laser system across tissue to maximize the examination area (top).
A diode-pumped solid-state (DPSS) laser (bottom). Advanced photoacoustic imaging systems have accelerated scanning times by employing DPSS lasers that generate pulses
under 5 ns, deliver output up to 20 µJ, and emit at key wavelengths precisely tuned to the absorption maxima of relevant tissue structures. Courtesy of iThera Medical.
For the tissues investigated, for example, a 7-ns laser pulse results in a low-pass filter with a cutoff frequency of 63 MHz — with the result that pulses in this range and above are broadened by this low-
pass behavior that, again, reduces resolution of the image. The DPSS laser emits pulses below 5 ns, which generate ultrasonic pulses with a cutoff frequency beyond 100 MHz. Thus, the laser ensures a wide detection bandwidth and a high spatial resolution of 10 µm axially and
40 µm laterally, down to depths of 2 to
3 mm.
To detect this broad ultrasonic frequency band, an RSOM system can use a spherically focused, piezoelectric broadband ultrasonic transducer able to detect acoustic signals with an ultrawide bandwidth over 100% at around a
50-MHz center frequency. During post-processing, the signals are split into multiple, color-coded frequency bands that allow clear visualization of separate structures of various sizes. Combined with the short pulse duration of the laser, this leads to a high intrinsic optical tissue contrast.
Conclusion and outlook
Multispectral photoacoustic imaging technology has reached clinical trials, where it has demonstrated many advantages over other methods. The noninvasive technique offers great penetration depth and high resolution. Laser sources that emit very short pulses with precisely matching wavelengths contribute significantly improved image data that can provide more information about the tissue under examination.
Further applications of the technology
include various preclinical research projects. For example, scientists at King’s College London implemented the 532-nm DPSS laser design for a miniaturized, ultrathin, high-speed endoscope intended for use with photoacoustic imaging in clinical diagnosis and surgical guidance2.

A representative illustration of the layout of DPSS lasers includes a fundamental pump laser (1064 nm) frequency doubled to a 532 nm beam, which then pumps a potassium gadolinium tungstate (KGW) crystal in an L-shaped cavity to produce first, second, and third Stokes outputs at 555, 579, and 606 nm, respectively. An acousto-optic tunable filter (AOTF) then routes one wavelength at a time to the output. SHG: second-harmonic generator. Courtesy of Elforlight.
Based on multimode fibers, this endoscope can be integrated within the cannula of a 20-gauge medical needle — including the ultrasound detector realized by using a single-mode optical fiber with a planoconcave microresonator. Shaping the wavefront by using a digital micromirror device enabled rapid raster scanning of a focused light spot at the
distal end of the multimode fiber for
tissue interrogation. The ultrathin
photoacoustic endomicroscopy probe
that this produced shows promise for guiding minimally invasive surgery by providing functional, molecular, and microstructural information of tissue in real time.
Meet the author
Keith Oakes is director of Elforlight Ltd., an AMS Technologies company, where he is responsible for laser development. He has over 40 years of experience as a laser physicist and has contributed to the design and development of a wide range of laser types.
References
1. M. Schwarz (2017). Multispectral optoacoustic dermoscopy: methods and
applications, https://mediatum.ub.tum.de.
2. Tianrui Zhao et al. (Aug. 1, 2022). Ultrathin, high-speed, all-optical photoacoustic
endomicroscopy probe for guiding
minimally invasive surgery. Biomed Opt Exp, Vol. 13, No. 8.