Scientists are using photonics to detect gravitational waves, the long-predicted phenomena that directly confirm Einstein’s general theory of relativity.
VALERIE C. COFFEY, SCIENCE WRITER
It’s an incredible story: Building the Laser Interferometer Gravitational-Wave Observatory (LIGO) took a collaboration of over 1000 people, some $500 million in funding from the National Science Foundation over three decades, and a lot of patience. But the vision paid off with observational evidence of one of the greatest mysteries in physics — the existence of gravitational waves.
In 2001, the first two LIGO facilities came online in the U.S. — one in Livingston, La., and the other in Hanford, Wash. — separated by 3000 km. The twin observatories are composed of giant underground tunnels in an L shape, each arm measuring 4 km long (Figure 1). At the end of each arm of the L, 200 W of light originating from a powerful NIR laser diode reflects back and forth between four of the smoothest fused silica glass mirrors ever known, called “test masses” and suspended as pendulums. The test masses create a Fabry-Pérot interferometer in each arm that together create a giant Michelson interferometer, awaiting miniscule disturbances that might indicate gravitational waves.
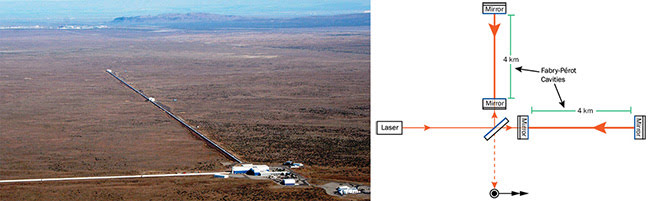
Figure 1. The Laser Interferometer Gravitational-Wave Observatory (LIGO) in Hanford, Wash., features two perpendicular arms measuring 4 km in length (left). Its twin observatory lies 3000 km away in Livingston, La. A basic Michelson interferometer with 4-km Fabry-Pérot cavities (right). Courtesy of Caltech/MIT/LIGO Lab.
As sometimes happens with scientific experiments, the observations of gravitational wave (GW) events at first went missing at LIGO. The biggest challenge to the detectors was avoiding false positives caused by spurious noise. For nine years, the research teams constantly strived to improve mirror alignment and upgrade the system, watching and waiting for a telltale signal, filtering out seismic vibrations coming from everything from earthquakes to passing trucks and faraway ocean waves. Researchers debated whether LIGO was sensitive enough to do its job. It wasn’t; not quite — even though the LIGO instruments could detect a variation in the laser beam’s fringe pattern on the order of a billionth of a billionth of a meter. But the complicated undertakings of design, alignment, control, and maintenance were immensely useful to engineers and scientists, who learned, among other things, how to make critical tweaks to the system design to improve sensitivity.
The upgraded system design encompassing those improvements, Advanced LIGO or aLIGO, took seven years to build. In 2015, the new and improved aLIGO detectors came online, with larger, heavier mirrors, a four-segment pendulum hung using silica glass fibers instead of a single segment hung with metal fibers, and an improved “active feedback,” real-time isolation system complementing the initial passive isolation system1.
The initial LIGO optics incorporated test masses of fused silica weighing 11 kg (22 lb) and measuring 25 cm (9.8 in.) in diameter. The updated test masses of aLIGO weigh a much heftier 40 kg (88 lb) and are 34 cm (13.4 in.) across (Figure 2). The new, highly reflective coating involves alternating dielectric layers of high-index, titania-doped tantala with low-index silica. The new design provided a tenfold improvement in sensitivity. The aLIGO detectors were locked down and ready to observe in mid-August 2015.
Figure 2. The 40-kg test masses of aLIGO are
polished to tolerances approaching 1 Å. The input and end mirrors are
suspended via silica glass fibers in a quadruple pendulum system that
isolates them from seismic noise. Courtesy of L. Hayashida/Caltech/MIT/LIGO Lab.
On September 14, 2015, less than two days after the improved aLIGO came online, the detector in Louisiana spiked, followed by a twitch in the Washington detector seven milliseconds later. The aLIGO detectors registered a signal in the hundreds-of-Hz frequency range, coincidentally within the low end of the human audio range. This “chirp” was the predicted signature of two inspiraling massive objects (Figure 3). The faint signal, which disturbed the laser beams by just a mere 10 to 18 m, confirmed that the irregular masses of two black holes had accelerated and collapsed together. The detector improvements had worked! After a period of confirmation, scientists announced GW150914, the first direct observation of gravitational waves ever. The GW150914 (named for the detection date) observation further confirmed Einstein’s 100-year-old theory of general relativity, which predicted that when massive objects collide, they create waves in the fabric of space-time.
Figure 3. A simulated inspiral gravitational
waveform of two rotating, massive objects behaves like a sinusoidal
wave, increasing in amplitude as they collapse together. If it weren’t
so faint, such a wave might sound like a low vibration sliding up in
pitch and ending in a chirp (a). The first gravitational wave detected
by the twin LIGO observatories was a 0.2-s “chirp,” the duration of
which reveals a black hole merger (b). Courtesy of A. Stuver/Caltech/MIT/LIGO Lab.
Over the next few years, the LIGO team observed several new black hole mergers, including the first detection by VIRGO, a sister observatory in Cascina, Italy. With 3-km-long arms, VIRGO came online in mid-2017 as a third potential witness of gravitational waves. Shortly thereafter, all three observatories confirmed a GW detection for the first time. Then, a watershed moment occurred. While signals of black hole mergers typically last only fractions of a second, an observation at the twin LIGO observatories on August 17, 2017, detected a distinctly different chirp lasting 100 seconds in the detection band. Team members had no doubt that such a signal must come from objects much less massive than black holes, as the rate of collapse decreases with lower mass objects. In a most fortuitous twist, LIGO had caught its first gravitational wave from merging neutron stars (Figure 4), an object designated GW1708172.
Figure 4. Two rotating neutron stars
spiral inward to become one in this illustration of GW170817, the event
that spawned gravitational waves coincident with observations of x-rays,
visible light, and gamma rays — one of the most important observations
in modern astronomy. Courtesy of NSF/LIGO/Sonoma State University/A. Simonnet.
Moments after the two stars collided, the Fermi Gamma-Ray Space Telescope detected a short gamma-ray burst (sGRB) in the same direction as the collapsing neutron star. Theories had predicted that collapsing neutron stars would create gamma rays, but the observation validated their relationship. Follow-up visible-light observations from other facilities confirmed a new pinprick of light in the same direction, at the edge of galaxy NGC 4993 some 130 million light-years away, showcasing a new era of astronomy (Figure 5).
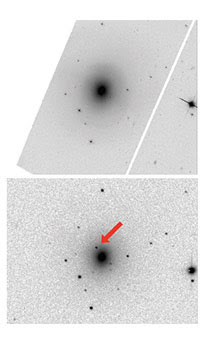
Figure 5. An image taken by Hubble
Space Telescope on April 28, 2017, of the galaxy NGC 4993. Bottom: An
arrow points to a new pinprick of light near the same galaxy, as imaged
by the Swope Telescope on August 17, 2017, just a few hours after other
observatories recorded gravitational-wave and gamma-ray events. This
short-lived fireball fits the model for a collapsed neutron binary pair
and is the first optical counterpart to a gravitional wave detection.
Swope Supernova Survey via UC Santa Cruz. Courtesy of Swope Supernova Survey via UC Santa Cruz.
Many more observations of the new object followed. And one after another, further observations of inspiraling binary black holes and binary neutron stars added up, combined with studies of co-located and simultaneous observations in UV, optical, and IR wavelengths.
The first wave of GW observations resulted in some 700 papers being published to date around the world.
What are gravitons?
In addition to confirming general relativity, LIGO has greatly contributed to our knowledge of gravitational events, black holes, and neutron stars. For example, the data confirms that collapsing neutron stars can act as a source of sGRBs and heavy metals. LIGO can potentially help answer many more remaining fundamental questions in physics, astrophysics, and cosmology, such as: Do gravitons, the theoretical particles that make up gravitational waves, exist? If so, what are their properties? How many gravitons are in a gravitational wave? What types of objects are formed when objects of different masses collide? Further observations of gravitational waves will be necessary to form a body of evidence large enough to quantitatively answer these questions. The LIGO team expects observations to grow as they execute improvements.
Future improvements are likely to involve longer interferometer arms, the observations of which could detect even fainter, more distant events, such as galaxy evolution and formation. One proposal described by LIGO Hanford staff scientist Sheila Dwyer suggests a detector with arms 40 km in length, which would enable studies back to the first stars in the universe.
Such projects with longer arms would require much larger test masses, which — according to GariLynn Billingley, aLIGO’s senior optical engineer at Caltech (Pasadena, Calif.) — would affect the heat absorption in the optics and consequently affect the choice of laser in the design.
A joint project between the European Space Agency and NASA, called the Laser Interferometer Space Antenna (LISA), would place three interferometers in orbit around the sun, set millions of kilometers apart in the convenient vacuum of space, to introduce more complicated locking of the distance and angles of the detectors. Improvement in one area of the detectors complicates other areas, but such a space-based system could study early galaxy formation — a prospect that makes astronomers salivate. In the meantime, scientists are hoping to remedy the simplest of LIGO’s limitations. For one, the sensitivity of the current LIGO instrumentation is limited by the coating thermal noise.
“The coating on the mirrors suffers from thermal energy transfer even at room temperature, affecting our detection range at our most sensitive point,” said Billingsley. A collaboration of coating experts led by Stanford University and the University of Florida have formed the Center for Coatings Research, an NSF-funded project, to examine how to mitigate the coating thermal noise and improve the rate of observation. The improvement will involve repolishing and recoating the test masses. The new coating design is expected to realize a reduction of almost a factor of two in coating thermal noise, which then increases the observation rate by 23, or a factor of eight.
Unlike typical photon detectors, GW detectors observe in all directions at once, even through the earth. According to David Shoemaker, spokesperson for the LIGO Scientific Collaboration and senior research scientist at MIT’s Kavli Institute for Astrophysics and Space Research, most astronomical observations measure electromagnetic radiation, the power of which falls off much more quickly as the light dissipates through the volume of space (1/r3). LIGO measures the amplitude of a wave, which falls off inversely to the distance to the source (1/r). “The beautiful thing about gravity waves,” said Shoemaker, “is that improving sensitivity by a factor of two means we triple the detection rate.”
The new coating is one of several projects focused on the next set of improvements to LIGO, called the A+ upgrade. Dwyer, one of the scientists on the upgrade, is focused on another aspect of the detectors: frequency-independent squeezing, an idea that uses an optical parametric oscillator (OPO) inside the vacuum chamber to reduce quantum noise. Explained Dwyer, quantum vacuum fluctuations from the photodetector cause two types of quantum noise that particularly limit sensitivity: radiation pressure noise at low frequencies and shot noise above 60 Hz.
“The quantum state of light can be thought of as a fuzzy ball,” said Dwyer. “The fuzz along one axis causes shot noise, the other causes radiation pressure noise. If we can squeeze the ball and make one axis shorter, we reduce one type of noise.”
The key to this quantum trick is the OPO, a Fabry-Pérot cavity containing a nonlinear crystal that enables energy exchange between different wavelengths of light. Reflecting the vacuum fluctuations off the OPO “squeezes” the shot noise, giving a higher signal-to-noise ratio at frequencies above 60 Hz, where neutron star mergers are detected. However, reducing the shot noise also causes an increase in radiation pressure noise at low frequencies. So Dwyer and colleagues are developing a 300-m-long filter cavity required to eliminate this additional radiation pressure (Figure 6).
Figure 6. Postdoc Terry McRae of Australian National University and LIGO Hanford, Wash., staff scientist Sheila Dwyer install stray light blocks on a suspended platform housing an optical parametric oscillator (OPO) that will be used to “squeeze light” in the next generation of LIGO sensitivity improvements. Courtesy Nutsinee Kijbunchoo (ANU/LIGO/Caltech/MIT).
The LIGO team estimates the next observing run with an improved LIGO will start in early 2019. The researchers hope to detect binary black hole inspirals every week or two, and binary neutron events every month or two. According to Shoemaker, such a higher rate of events would enable LIGO to move from a regime where rare events can only begin to cleave the rough edges of a theory, into the realm of astronomy where robust models are based on statistics.
“We can do a lot more than just collect neutron binaries like butterflies,” Shoemaker said. “The real promise is to actually probe the fundamental properties of space-time to form deeper conclusions about its structure and how general relativity works on a large scale.”
References
1. J. Aasi et al. (2015). Advanced LIGO: LIGO scientific collaboration. Class and Quantum Grav, Vol. 32, Issue 7, 074001, https://doi.org/10.1088/0264-9381/32/7/074001.
2. B.P. Abbott et al. (2017). GW170817: Observation of gravitational waves from a binary neutron star inspiral. Phys Rev Lett, Vol. 119, 161101, https://doi.org/10.1103/PhysRevLett.119.161101.