With a number of recent demonstration successes and growing worldwide interest, free-space optical communications is set to transform the global and extra-global telecommunications landscape.
DON M. BOROSON, MIT LINCOLN LABORATORY
Back in the early 1960s, when first the laser and soon thereafter the semiconductor laser were invented, it was predicted by visionaries that, before long, such sources of coherent light would be used for communication. It actually took more than 25 years, but by the late ’80s, there had been enough invention and development that optical fiber telecommunications was on its way to becoming the world’s primary means of long distance terrestrial communications. Free-space optical communications engineers thought they’d follow close behind, but it has actually taken another few decades to achieve a critical mass of system concepts and technologies. With these in hand, developers around the world have now demonstrated all the most challenging parts of the technology, and we can now state that free-space optical communications is finally taking hold in a number of classical and novel markets.

NASA’s in-development Laser Communication Relay Demonstration (LCRD)
plans to include two GEO terminals, two ground terminals and an LEO
terminal.NASA’s in-development Laser Communication Relay Demonstration (LCRD) plans to include two GEO terminals, two ground terminals and an LEO terminal. Courtesy of NASA.
Higher data rates, smaller terminals
Optical wavelengths are more than four orders of magnitude shorter than those of even the highest practical radio frequencies. One result of this is that the useable bands are multiple terahertz wide (Figure 1) as opposed to gigahertz wide.
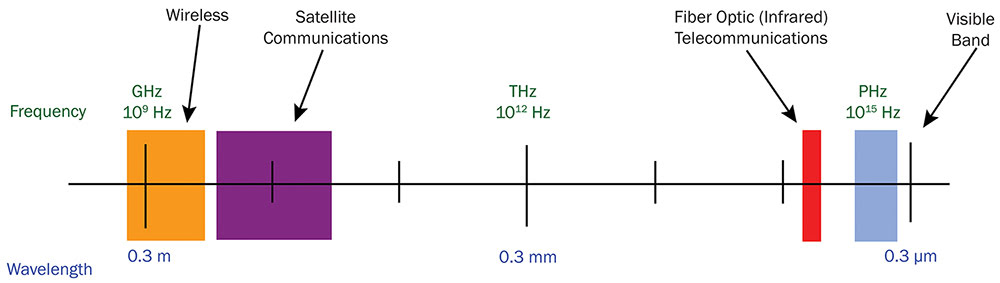
Figure 1. An overview of the communication bands on the spectrum. Courtesy of MIT Lincoln Laboratory.
Such wide bandwidths suggest that optical signals have the potential to support huge rates of data transfer. Furthermore, because even these wide bandwidths subtend only small fractional bandwidths, single optical systems can be designed that can efficiently process, transmit and receive huge numbers of wavelength-multiplexed communications signals.
Additionally, short wavelengths mean that the optical antenna (i.e., telescope) gain — which is proportional to (D/λ)2for telescope diameter D and wavelength λ — from small apertures can be many millions of times greater than from even large radio dishes. As an example, it would take a dish over 2 km in diameter at X-band to create the same gain as a 10-cm telescope at 1.5 µm.
Such narrow beams have multiple useful properties: They can deliver power very efficiently over long distances, potentially enough to support the desired high data rates, and they are difficult to either intercept or disturb, providing intrinsic security. Additionally, their receiver fields of view are usually designed to be so narrow that it is nearly impossible to be bothered by transmissions from other users, thus almost completely eliminating the need for any kind of frequency coordination or registry. In fact, at present, free-space optical bands have no regulations other than for safety.
Of course, it is also well-known that a laser beam cannot penetrate walls, hills, clouds or dense fog — nor does it bend around corners without reflective assistance. This inherent limitation means that laser communications will likely never achieve the truly universal utility of the various radio bands. Nevertheless, communications system architectures designed to have multiple connectivity options or the ability to wait for service do exist, and laser communications can often meet and surpass their needs quite well. For instance, above-the-weather connections or space-to-ground-network systems are already being implemented.
Another first-principles drawback is that, because photons are more energetic than radio waves, there is proportionally more “quantum noise” in optical receivers. What this means is that the signal-to-noise ratio (SNR) is transmitted with an efficiency more like the smaller (D2/λ), although this is still over four orders of magnitude greater than for radio systems. It also means that optical systems with omni characteristics are not that power efficient, and thus may not be able to support high data rates. On the other hand, photons are energetic enough that they can be detected singly, noiselessly and with high probability, allowing for receiver architectures that are much more efficient than those for radio.
For the most part, free-space links that are being proposed have at least one end flying in an aircraft or a spacecraft. Nevertheless, there are many communication system architectures where such placement makes sense.
Components suited to a range of architectural requirements
Although there were successful atmospheric and airplane-based demonstrations in the 1970s, and attempts at space designs in the 1980s, it was not until the late 1980s and 1990s that there started to be enough flight-worthy components and subsystems available so that designers could build laser communication terminals meeting the needs of the desired air- or space-based optical capabilities. Very briefly, the main components unique to such systems include optical transmitters and optical receivers; space-worthy, high-performance steerable telescopes; and the means for finding and stabilizing the narrow beams.
There are several possibilities for each of these subsystems, depending on the goals of the complete communications system. Factors that lead to specific technology selections include desired data rate; terminal mass and electrical power; required speed of link initiation; robustness to a variety of conditions; link range and motions of the terminals, atmosphere or not; and desired system complexity and cost. Thus, there is not a one-size-fits-all design.
Furthermore, until the free-space laser communication industry grows, most components will have been initially developed by other markets, or will have been specially designed, built and flight-tested in small quantities, often at high cost. Even so, there are presently enough components such that almost any architectural need can be fulfilled, with economies of scale not far off.
Transmitters transformed
Suitable transmitter systems of the 1980s often made use of semiconductor laser diodes — whose development was greatly driven by the compact disc industry. These diodes produced only a few tens of milliwatts. However, it was the invention and development of the doped-
fiber amplifier in the late 1980s, along with the realization that fiber-coupled modules were feasible for free-space transmitters and receivers and could greatly simplify space- or air-based systems, that the field really started to advance. Shortly thereafter, many system designers turned to the 1.55-µm fiber telecom band or the related 1.064-µm band, allowing incorporation of components developed by the huge fiber telecommunications and subsidiary markets. In fact, in the present day, terrestrial components are shrinking as a result of integrated photonics, and there is no doubt that these advances will find a place in the free-space community. (The 1- to 1.5-µm bands will continue to dominate the high data rate free-space markets, but there will also be specialty niches for 0.8 to 0.9-µm systems as well as visible-band and even UV.)
Receivers: a choice between high data rates and photon efficiencies
The choice of a receiver is usually driven by the desire to achieve either extremely high data rates or very high photon efficiencies. The latter objective allows the designer to keep the telescopes small, and laser power low.
For the highest bandwidth formats, detectors need to be physically small or even single-spatial-mode, which puts special constraints on receivers in the atmosphere because of turbulence, both naturally occurring and exacerbated by aircraft moving through the air. Adaptive optics, using either deformable mirrors or coherent array concepts, can flatten out the received wavefronts and enable coupling of the light into the single-mode detection systems. Such single-mode receivers include coherent or optically preamplified architectures, both being quite mature. Also, similar to some radio frequency systems, non-demodulating transponders are feasible, based on single-mode reception and amplification.
Some receivers, such as avalanche photodiode detectors, are of particularly low complexity and do not require single-mode reception. They are usually 10 to 20 dB less efficient, but such performance may be adequate for some system designs.
Finally, photon-counting detectors — semiconductor, photo-multiplier tubes or superconducting — have been shown to provide up to 5- to 15-dB better photon efficiency than coherent receivers. As an added benefit, they do not necessarily require single spatial mode reception. At present, though, most of these require more complex support systems such as cryo-refrigerators, and so are relegated to ground uses. There is no doubt, though, that because of their superlative efficiency, future developments will lead to such receivers being flyable in small aircraft or even in space.
Flight optics and pointing, acquisition and tracking systems
Large steering motions of the beam can be implemented by gimbaling the telescope or by steering the beam at the telescope’s entrance using periscopic mirrors or Risley prisms. Small beam motions can be achieved either with these same approaches or with beam steerers — mechanical steering mirrors or solid-state devices — in the small-beam-space behind an afocal telescope.
Beam pointing is initially based on direction estimates provided by combinations of star trackers, Earth sensors, GPS and gyros. Even the best of these, though, usually have errors much larger than the beamwidth provided by the telescope (usually only tens of microradians). Therefore, a link spatial acquisition phase is almost always required, where the two terminals find each other. Here, too, there are multiple possibilities: transmitter scanning or broadening and/or special receiver sensors for detecting signals from a wide field of view, all as prescribed by an agreed-to protocol.
Because the beams are so narrow, the designer must also take into account the relative cross-velocity of the two platforms, which results in needing to “point-ahead” the transmitter in front of the receiver. Angles for this range from 17 urad for geostationary orbit (GEO) to ground, to approximately 60 urad for GEO to LEO (low Earth orbit), and up to as much as 500 urad for inter-planetary systems.
Once the beams are correctly pointed, they then need to be kept stable to a small fraction of their width. Active tracking designs based on the incoming beam require a means of detecting the motion using a special detector or a conically scanned receiver, as well as a mechanism such as a small, fast mirror, behind the telescope. Also possible are non-received-beam techniques such as inertial references or actively stabilized platforms.
State of the industry and future deployments
Despite the many architectural possibilities, and seeming complexity, it is fair to say that, at this date, each of these techniques has been demonstrated many times, some even in the several successful space and air demonstrations that have occurred. Thus, designers can choose architectures to meet specific system needs.
Standardization is also now being addressed by the community. For instance, the international space industry (The Consultative Committee for Space Data Systems [CCSDS]) is presently in the process of proposing interoperability standards for signaling.
With many architectural possibilities, more and more components becoming available, and with subsystem and system costs dropping, there is no doubt that the free-space optical communications industry is on the verge of becoming a major player in a number of classes of global and extra-global telecommunication. The high performance and small terminal size will likely even enable as-yet uninvented new architectures of space and air connectivity.
Meet the author
Don Boroson is a laboratory fellow at the MIT Lincoln Laboratory, where he has been developing advanced free-space laser communications systems for many years. He was the principal investigator for NASA’s recent Lunar Laser Communication Demonstration.
Breakthroughs in Free-Space Systems
Terrestrial links: Fixed terrestrial links have been constructed and marketed for years, being made reliable by keeping the links relatively short.
GEO relay systems: Geostationary orbit (GEO) relays servicing few-Gbps users include the European Data Relay System (EDRS) of the European Space Agency and Airbus, which has already launched and is operating two GEO and four low Earth orbit (LEO) user terminals in orbit (Figure 2); NASA’s in-development Laser Communication Relay Demonstration (LCRD), to include two GEO terminals, two ground terminals and an LEO terminal (see opening image); and the similar Japanese Data Relay System (JDRS), both of the latter two scheduled for launch in the next few years.

Figure 2. The European Data Relay System (EDRS) is a public-private partnership between the European Space Agency (ESA) and Airbus Defence and Space. It uses advanced laser technology to relay information collected by lower orbiting satellites to the Earth via geostationary nodes. Courtesy of ESA.
Crosslink: In 2008, an internationally coordinated crosslink between two LEO satellites — the U.S. Missile Defense Agency’s NFIRE and the German Aerospace Center’s TerraSAR-X — was accomplished.
Aircraft: Aircraft-based optical commununication has been demonstrated by various players including the Defense Advanced Research Projects Agency (DARPA), the German Aerospace Center (DLR), the U.S. Department of Defense, Facebook, MIT Lincoln Laboratory and others. Project Loon, with a system housed on a stratospheric balloon, was also recently demonstrated by Alphabet’s innovation lab, X (Figure 3).
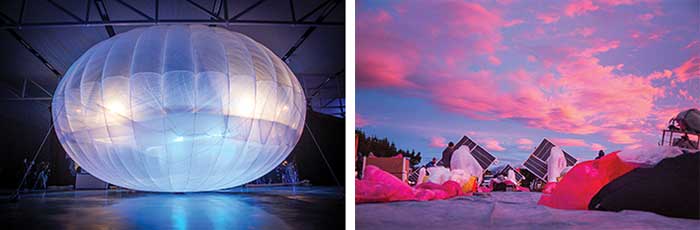
Figure 3. An optical communication system housed on a stratospheric balloon (left), part of Project Loon, was demonstrated in New Zealand in 2013 by X, an innovation lab of Alphabet. Project Loon prepares to launch in New Zealand, 2013 (right). Courtesy of Project Loon.
LEO-based Direct-to-Earth: A number of small (or even CubeSat) satellites are demonstrating laser commmunications capabilities at up to a few Gbps. Japanese, German, U.S. and even student-based teams are all experimenting with this capability.
Deep-Space-to-Ground: NASA recently announced that the Jet Propulsion Laboratory’s Deep Space Optical Communications (DSOC) system will fly on the Psyche spacecraft in 2023; and until recently, ESA was planning on optical communication from its Asteroid Impact Mission (AIM). Similar to these was the recent highly successful NASA/MIT Lincoln Lab demonstration — the Lunar Laser Communication Demonstration (LLCD) — of duplex high-rate communication from a lunar-orbiting spacecraft, LADEE, to ground terminals, where inertial stabilization, high-efficiency photon-counting receivers, and reliable error-free through-the-atmosphere communication were demonstrated at up to 622 Mbps on the downlink and 20 Mbps on the uplink (Figure 4).

Figure 4. Artist’s rendering of NASA’s Lunar Atmosphere and Dust Environment Explorer (LADEE)
Demonstrating Lunar Laser Communications Demonstration (LLCD). Courtesy of NASA.