David Leuenberger, Optotune AG, and Fabian F. Voigt, University of Zurich
Focus-tunable lenses speed up and enhance three-dimensional imaging capabilities in a diverse set of modern microscopy methods including confocal, two-photon and light-sheet microscopy.
Microscopy beginners may be perplexed when they notice that parts of a sample that appear only marginally out of focus often look much more blurred in an image. The depth of field as seen by the human eye seems to be considerably larger than the depth of field seen by a camera. This bewildering effect occurs because the eye accommodates: While looking through a microscope, the user constantly – and often unconsciously – shifts the plane of focus without touching the focus knob, simply by tuning the eye lenses to a different focal length. Tunable lenses have thus helped researchers gain a more intuitive feel for the three-dimensional shape and texture of microscopic objects since the invention of microscopy.
Implementing a similar device in modern microscopes with electronic image acquisition is highly desirable. Today scientists have an increasing need to image the structures and functions of living organisms at high spatial resolution on shorter and shorter timescales. Modern biological microscopy is also slowly moving away from imaging small samples, flattened between a slide and coverslip, toward 3-D cell cultures, whole embryos and even in vivo imaging of animals to study development and physiology in more natural environments.
Traditionally, acquiring 3-D imaging data required mechanical translation of either the objective or the sample with a stage or a piezoelectric objective Z-scanner. Because of the mechanical inertia of moving parts in such devices, achieving volume-scan rates above 10 to 20 Hz for a Z-range of hundreds of microns is highly challenging.
An alternative solution called “remote focusing” involves changing the convergence of light as it enters or exits the microscope objective to induce an axial shift of the excitation or emission focus, respectively. All kinds of tunable optical elements can be used for this purpose: spatial light modulators, deformable mirrors and focus-tunable lenses, for example. With their low cost, simple construction and control, and wide focus-tuning ranges, focus-tunable lenses are especially well suited for microscopy applications demanding fast volume sampling at moderate resolution.
Tunable-lens technology
Optotune’s shape-changing lens is based on elastic polymer materials. The core element of the lens consists of a thin membrane that builds an interface between a liquid-filled chamber and air (Figure 1). To tune focal length, a voice coil actuator exerts pressure on a liquid reservoir surrounding the clear aperture of the lens. Fluid is thus forced into the center of the lens, changing the curvature of the membrane. Controlling the electrically tunable lens (ETL) is straightforward, requiring only an off-the-shelf current controller or lens driver to supply the lens with a current between 0 and 290 mA.
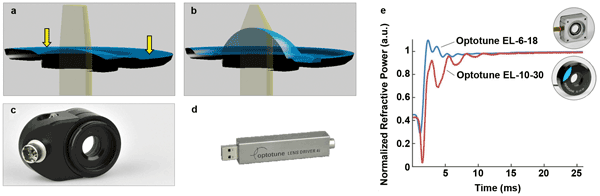
Figure 1. (a, b): Working principle of Optotune’s electrically tunable lens (ETL). A current-controlled electromagnetic or mechanical actuator pushes down on the liquid-filled lens container, forcing the lens liquid into the center of the lens and changing its shape. (c) For the ETL, Optotune offers a software-controlled lens driver (d) that supplies the electrical current. (e) Typical response time of Optotune’s ETL is on the order of 5 ms. The actual settling time varies with lens aperture. Courtesy of Optotune.
A wide range of ETLs is available with apertures ranging from 6 to 16 mm. Typical focal-length ranges are 52 to 120 mm or 80 to 200 mm for versions with high- and low-dispersion lens liquids, respectively. During operation, the control current can heat up the lens, resulting in a temperature-dependent focus drift. Because the thermal focal-length expansion of a liquid lens is about two orders of magnitude larger than that of a glass lens, lenses need integrated temperature sensors. Combined with a temperature sensor close to the liquid, as well as storage of the calibration curve on the lens, USB driver firmware is allowed to calculate the correct current value to set and maintain the lens at a given focal power.
A big advantage of ETLs is the short response time of a few milliseconds. Figure 1e shows typical examples of the normalized refractive power as a function of time in response to a rectangular step impulse. The tunable lenses offer a large transmission over the range of 240 to 2500 nm and high damage thresholds (10 kW/cm2 for continuous-wave operation at 1064 nm), and they are polarization-maintaining.
Integrating a tunable lens in a microscope
Modern microscopes use infinity-corrected objectives, which means that light originating in the sample emerges from the objective as a parallel beam. To create an image, an additional tube lens is required (Figure 2). Conversely, by sending a collimated laser beam into the objective, the laser light is concentrated to a focus inside the sample. Changing the collimation state of the beam with a tunable lens shifts the focal plane. For example, when sending a diverging beam into the objective, the focus is shifted away from its front lens.
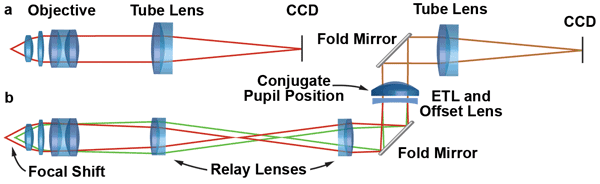
Figure 2. (a) In a microscope with infinity-corrected optics, the image is formed by the objective and tube lens. (b) By inserting an additional relay system composed of two achromats between the objective and the tube lens, a conjugate pupil is formed. The ETL and the offset lens can be placed at this position for axial focusing without a change in numerical aperture or magnification. Placing the ETL and offset lens horizontally avoids gravity-induced distortions of the lens membrane. Courtesy of Fabian F. Voigt.
For most 3-D microscopy applications, the ability to both increase and decrease the working distance of the objective is required. Some ETLs are restricted to tuning between positive focal-length limits. In this case, pairing them with a fixed negative offset lens (OL) is necessary to change the beam from convergent to divergent.
When looking through the eyepieces of a microscope, human observers move their heads until their eyes are positioned at the microscope’s exit pupils, often visible as little bright disks that seem to hover above the eyepieces. When placed at exit pupils, eyes have the best overview of the microscopic image and perform best as “integrated” human focusing devices. Ideally the ETL/OL combination is placed at such a pupil position as well, but using the exit pupil of standard eye-
pieces is not normally beneficial: The high intermediate magnification of typical tube-lens-and-eyepiece combinations drastically restricts the available focusing range. This is because the tuning range is inversely proportional to the square of the microscope’s magnification.
A better option is to create a pupil-position conjugate to the microscope objective with a custom-built relay system (Figure 2). Care is needed to place the ETL/OL assembly in a vertical portion of the optical path (Figure 2b). Otherwise the images can exhibit unwanted aberrations (especially coma) due to gravity-induced deformations of the lens membrane. In newer generations of research microscopes with very modular designs, integrating such a relay system is usually straightforward.
Nonetheless, some commercial instruments are not supposed to be modified extensively by users. In this case, the ETL/OL combination can be placed at other positions along a parallel beam path. The drawback is that placing a tunable element far away from a conjugate pupil will lead to a change in magnification and numerical aperture (NA) while focusing. In optical design parlance, the system is not telecentric anymore. A focal shift is thus accompanied by a zoom effect and change in resolution. For small tuning ranges – a few tenths of a micron – these effects are usually very small and tolerable. For larger focus displacements, the magnification change can be compensated for by image processing.
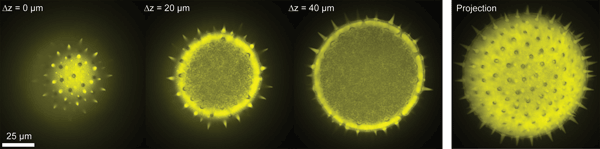
Figure 3. Z-stack of a pollen grain (100-µm diameter) taken with a 40× objective and an ETL integrated into a spinning-disk confocal microscope. Single frames at different axial focus positions and a maximum-intensity projection are shown. The maximum Z-range was 60 µm. Courtesy of Fabian F. Voigt and Helge Ewers.
In designs for remote-focusing systems, a significant trade-off exists between cost and complexity. Unlike deformable mirrors or spatial light modulators, most tunable lenses have only a single degree of freedom, namely the focal length. A microscope equipped with a single ETL in a remote-focusing path cannot perform the same sophisticated aberration corrections over the Z-tuning range as a true adaptive optics microscope. However, focusing with an ETL comes at a tiny fraction of the cost of a fully fledged adaptive optics setup.
Application example: Confocal microscopy
One of the most important microscopy techniques is confocal microscopy, with its large variety of applications in cell biology, single-molecule physics and many other disciplines. We tested an ETL in combination with a commercial spinning-disk confocal microscope module (Yokogawa CSU-X1) attached to an inverted microscope stand (Olympus IX71).
As it was impossible to integrate a relay system in this microscope, the ETL/OL assembly was mounted in a custom filter cube that could be rotated into the light path. With a 40× objective and 1.3 NA (Olympus UPLFLN 40XO), a maximum Z-range of 60 µm was achieved.
Application example: In vivo two-photon microscopy
Because of its excellent imaging capability in scattering media, two-photon excitation is a technique well suited for fluorescence imaging deep inside brain tissue. Combined with functional indicators of neuronal activity and in vivo imaging protocols, two-photon microscopy is a standard method for recording population activity ranging from tenths to hundreds of neurons that are hundreds of microns deep inside the living mouse brain.
Sampling a single focal plane provides only a glimpse of the distributed activity patterns in local networks. Therefore, we modified an existing two-photon microscope capable of very fast X-/Y-scanning with acousto-optical deflectors with an ETL and OL.1 The acousto-optical deflectors can rapidly target a femtosecond laser beam to a large number of neurons in a random-access pattern at kilohertz rates.
Adding the ETL enabled rapid targeting of several focal planes. We achieved imaging rates of 30 to 40 Hz for up to 40 neurons spread across two planes separated by 30 to 100 µm (Figure 4). Apart from multiplane imaging, the ETL also can be used for motion correction in awake and behaving animals.2
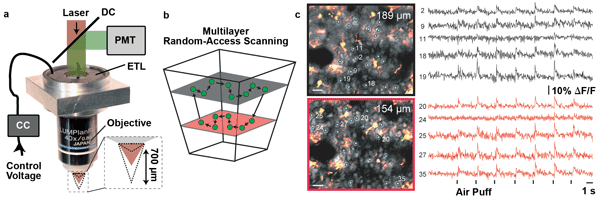
Figure 4. (a) Objective mount for integrating an ETL into a custom two-photon microscope with acousto-optical deflectors for in vivo imaging of neuronal activity in the mouse brain. The ETL is driven by a current controller (CC) and mounted close to the objective in combination with an offset lens (not shown). (b) As the ETL is not placed in a conjugate pupil position, the scanned volume has a tapered shape. The acousto-optical deflector microscope can rapidly measure neuronal activity with a calcium indicator in a random-access scan pattern. The ETL adds the ability to image multiple planes at rates up to 40 Hz. (c) These images show two planes at different depths (neurons shown in gray and astrocytes in orange). The cells selected for fast calcium imaging (1 to 40) are marked. The neuronal signals exhibit responses stimulus-locked to repeated air puffs applied to the whiskers of the animal. Scale bars: 20 µm. Courtesy of Benjamin Grewe, Fabian F. Voigt and Fritjof Helmchen.
Application example:Rapid 3-D light-sheet microscopy
Within the past decade, light-sheet microscopy and selective plane illumination microscopy (SPIM) have become recognized as ideal tools for in vivo imaging of biological specimens (e.g., for recording embryonic development in zebra fish and in fruit flies). In SPIM, the sample is illuminated from the side with a light sheet to restrict the excitation of fluorescence to a single plane in the focus of a microscope objective. SPIM offers excellent optical sectioning, low phototoxicity and high image-acquisition speed, and was named 2014 Method of the Year by Nature Methods.3
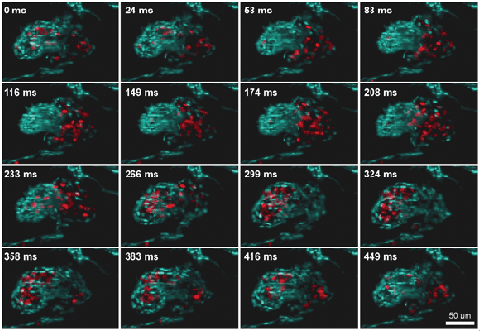
Figure 5. 3-D reconstruction of a beating zebra fish heart imaged at a 60-Hz volume rate with a light-sheet microscope equipped with an ETL for fast Z-scanning (ETL-SPIM). The heart is labeled in blue; red blood cells are shown in red. Courtesy of Florian Fahrbach, Michaela Mickoleit and Jan Huisken.
Recently a SPIM setup has been equipped with an ETL for fast volume scanning. The use of cameras running at hundreds to thousands of frames per second enabled the acquisition of 10 to 20 planes within a beating zebra fish heart at an unprecedented rate of 30 to 60 volume scans per second.4 Such high volume rates allow the tracking of single red blood cells flowing through a beating heart.5
Looking ahead
In the future, ETLs with larger clear aperture and tuning range will be available, along with innovative driving electronics that will improve repeatability by an order of magnitude. It is also conceivable that microscope objectives with integrated tunable lenses will become available for routine use over the next decade.
Meet the authors
Fabian F. Voigt is a doctoral student at the Brain Research Institute, University of Zurich, Switzerland; email: [email protected]. Dr. David Leuenberger is sales manager at Optotune AG in Dietikon, Switzerland; email: [email protected].
Acknowledgments
The authors thank Manuel Aschwanden, Jerry Chen, Adriana Dabacan, Helge Ewers, Florian Fahrbach, Benjamin Grewe, Fritjof Helmchen, Jan Huisken, Michaela Mickoleit, Oliver Pfäffli and Mark Ventura for their contributions to making fast 3-D microscopy with ETLs possible.
References
1. B. Grewe et al (2011). Fast two-layer two-photon imaging of neuronal cell populations using an electrically tunable lens. Biomed Opt Express, pp. 2035-2043.
2. J. Chen et al (2013). Online correction of licking-induced brain motion during two-photo imaging with tunable lens. J Physiol, pp. 4689-4698.
3. E.H.K. Stelzer (2015). Light-sheet fluorescence microscopy for quantitative biology. Nat Methods, Vol. 12, Issue 1, pp. 23-26.
4. F. Fahrbach et al (2013). Rapid 3-D light-sheet microscopy with a tunable lens. Opt Express, pp. 21010-21026.
5. M. Mickoleit et al (2014). High-resolution reconstruction of the beating zebrafish heart. Nat Methods, Vol. 11, Issue 9, pp. 919-922.