Full company details
Excelitas Technologies Corp.
Corporate Headquarters
2545 Railroad St., Ste. 300
Pittsburgh, PA 15222
United States
How Single-Photon Detection Powers Countless Applications
Photonics Spectra
Feb 2022The number of ways to detect and count single photons is growing, and applications for single-photon detection are expanding and emerging at a similar pace.RICHARD SIMONS, EXCELITAS TECHNOLOGIES
The technologies we use in our everyday activities to monitor the world around us — to develop new medicines or to produce the materials we use to build homes, offices, and factories — all rely on the most precise measurements of key parameters possible. As the need for accuracy and precision has increased, optical measurement methods have increasingly become the go-to solution to getting the information we need. More specifically, single-photon detection has become increasingly critical to helping us reach required performance levels.
Today, single-photon detection enables a variety of applications. It enables particle sizing for industrial process control, enhances fluorescence detection and lifetime measurements in life sciences research, and improves flow cytometry’s detection of nanoparticles. Single-photon counting also augments the ability of lidar and range-finding systems to support applications ranging from wind measurement to air monitoring for atmospheric aerosols, and from pollutants to helping cellphone cameras find their focus.
Single-photon detection will also enable many future applications, including
helping to increase lidar range for autonomous vehicles while ensuring the laser source remains eye safe. It will help to secure communications by detecting the single photons used for ones and zeros
in quantum key distribution, and by detecting entangled photons and calibrating the single-photon generators needed. And in quantum computing, it will help by detecting the single photons used to generate or interrogate qubits.
These current and future developments all rely on continued advancements in the science behind some of these applications. But it is clear that single-photon counting will help these and future technologies.
Particle sizing
One of the most common applications for single-photon counting has been particle sizing based on dynamic light scattering, a well-established technique used to
measure the size and distribution of particles, emulsions, or molecules within a liquid. Such particles move randomly as they collide with the liquid’s molecules. This process is known as Brownian motion, named after Robert Brown, a botanist who in 1827 used a microscope to observe the motion of pollen in water.
One of Albert Einstein’s first major scientific contributions was to model the particle behavior described by Brown. Einstein understood that a pollen particle suspended in water would be struck by water molecules coming from various directions, per Brownian motion. But Einstein realized that, when strikes were measured over very short timescales, unequal numbers of strikes would come from each direction. Therefore, within short timescales, there would be a net
momentum change for the particle, and the particle would also change direction.
NASA’s Cloud-Aerosol Transport System (CATS) lidar, which is mounted on the International Space Station, relies on a single-photon avalanche photodiode (APD) to detect return signals from atmospheric aerosols and clouds. Courtesy of Excelitas Technologies.
Momentum is the product of mass and velocity. So, for any given change in momentum, a larger particle would experience a smaller change in velocity.
Dynamic light scattering measures the variations in intensity of light that is scattered from particles suspended in a liquid. As particles get smaller, the amount of light they scatter declines until a single-photon detector is needed to measure the intensity variations and, from there, the particle size.
Dynamic light scattering is used to characterize particles or molecules measuring from under 1 nm to over 10 µm, which allows measurement of proteins, nanoparticles, polymers, and emulsions and enables technologies such as drug discovery, paint production, 3D printing, and many others.
For many years, the ideal light source for dynamic light-scattering instruments has been the helium-neon (HeNe) laser, which emits light at 633 nm. The ideal detector to accompany this laser has been a silicon avalanche photodiode (APD), which operates in what is known as Geiger mode because the detector operates in a manner analogous to a Geiger counter. Namely, it is poised in an unstable state to detect single photons, but then it needs to be quenched and reset to detect subsequent photons. APD detectors are well suited to this application compared to photomultiplier tubes, due to the much higher photon-detection efficiency that APDs offer at the prescribed wavelength range.
Lidar and range-finding
Lidar and range-finding both detect the scattering or reflection of light from particles some distance away from the source and detector. Range-finding typically measures the distance of a single target, while lidar maps objects within a 3D volume at a distance.
Particle sizing is one of the most common applications for single-photon counting, where dynamic light scattering is used to measure the size and distribution of particles, emulsions, or molecules within a liquid. Smaller particles exhibit more motion and more frequent directional changes. Shining laser light on the particles can help to characterize, count, and analyze them based on how the light scatters in terms of frequency and intensity. Courtesy of Excelitas Technologies.
Time-of-flight (TOF) detection, which is straightforward in principle but challenging at long and short distances, measures the time it takes for a light pulse to reach a target and return some proportion of its photons as scattered or reflected light. The round-trip time divided by the speed of light in the medium (usually air, but sometimes the vacuum of space) discloses the round-trip path length to the target. The accuracy of the TOF distance measurement is governed by a combination of the laser’s pulse width and the timing resolution of the system’s detector.
Simple diode lasers and fast APDs operating in linear mode can easily provide the necessary accuracy for many lidar or range-finding systems, including those used by surveyors or golfers. In these applications, the distances involved, the time available to take a measurement, and the reflectivity of the target permit many photons to return to the detector. Detection of single photons is not needed.
The automotive lidar systems being designed to enable autonomous vehicles, however, demand greater performance, eye safety, and reliability while minimizing costs. The autonomous vehicle market is still developing, and automakers are exploring many different lidar technologies to provide short- and longer-range mapping of the space around the vehicle. The technical challenges of spotting an object on the road in time to allow the vehicle to safely respond are amplified by contrasting needs. The lidar system needs to detect hard-to-see obstacles as far ahead as possible and yet limit the laser output to within eye-safety limits.
One solution to balancing these demands is to employ an eye-safe laser wavelength to allow higher laser output powers. A downside to this approach is that the costs for the lasers and the detectors used for longer wavelength operation are comparatively higher. An alternative solution to increasing laser output is to increase the sensitivity of the detector, which — given the performance demands for automotive lidar — favors use of single-photon detection.
Lidar systems installed on orbiting spacecraft also use single-photon detection to enable the measurement of polar ice levels, sea levels, vegetation at Earth’s surface, and cloud height. Spaceborne systems measuring the canopy height of forested areas are possible using lasers with pulse widths in the 10-ns range. Detection requires internal jitter to be well under 1 ns, between the emission of the output signal and the absorption of the photon in the canopy.
In such demanding applications, the use of APDs in Geiger mode is again predicated by their superior photon detection efficiency. Their ruggedness is also a factor, as they must perform reliably after undergoing the shock and vibration of launch followed by the harsh environment of space. APDs offer excellent resistance
to efficiency degradation versus photomultiplier tubes and can withstand accidental exposure to relatively high light levels.
The detector in time-of-flight lidar systems can be a simple PIN (positive-intrinsic-negative) photodiode when the system is targeting photon-rich environments. More typical applications may require an APD detector operating in linear mode. Detecting photonic signals from poorly reflecting or extremely distant targets, however, calls for more-sensitive single-photon detectors. Courtesy of Excelitas Technologies.
Lidar is also used in ground-based applications to capture accurate remote measurements of aerosols and other particulates in the atmosphere. Such applications involve long detection distances and a low return-signal probability. Using single-photon detectors to detect the backscatter from laser pulses, however, enables real-time measurement of atmospheric information.
Aerosols can be some of the most harmful air pollutants. Being able to measure their concentration and movement in the atmosphere is key to predicting air quality in cities, measuring emission plumes from smokestacks to enforce environmental regulations, and enabling surface mining operations to accurately determine when wind conditions are transporting dust into sensitive areas.
Single-photon lidar can also be used to measure natural aerosols to gather information about wind patterns. Such information is useful for planning where to build wind farms and when to shut them down in anticipation of high winds. It also provides insights into atmospheric stratification to improve weather forecasting. Single-photon lidar systems can also be quickly deployed to monitor volcanic ash plumes and to provide accurate data to optimize aviation safety while minimizing flight cancellations and delays.
Many of these lidar and range-finding applications are not typically used in cost-sensitive markets. Such applications often rely on high-performance single-channel detectors to meet system requirements. These detectors are made using traditional APD manufacturing methods and the highest-quality materials.
For automotive applications, lidar
single-channel detectors are fine in terms of performance but less attractive cost-wise when compared to other options. High-volume CMOS manufacturing techniques offer a lower-cost alternative, however. These chips incorporate the detection layer and the initial signal processing and control electronics into multiple layers of a single substrate, massively reducing the cost of the single-photon detector. The CMOS detector will be less efficient and more noisy than traditional APDs, but its performance is good enough to increase the range of lidar systems. CMOS detectors also allow 2D arrays and eliminate the need for complex scanning laser systems — further reducing cost.
CMOS-compatible single-photon avalanche diodes assembled as 2D arrays are being used in even more cost-sensitive markets by further reducing operating distance. Cellphone designs, for example, are incorporating these devices as highly sensitive proximity sensors that can operate with low photon incidence. TOF cameras with megapixel resolution are also being developed to allow the acquisition of 3D images at far-higher-than-video frame rates, enabling augmented reality applications.
Fluorescence measurement
The fluorescent lighting that for years relied on fluorescent tubes, and more recently on LED lamps, converts UV or blue light into longer wavelengths to create a white-light output. Measurement of fluorescence is another area in which single-photon detection enables improved capabilities, particularly in biochemistry and medicine, where fluorescence is used to track and analyze biological molecules or processes by monitoring fluorescent emissions. Measuring the fluorescence of biological molecules or processes is also critical to biochemistry and medicine. Tagging cellular components with specific fluorophores allows the visualization and study of specific areas within a sample.
The use of single-photon detection enables fluorescence lifetime imaging microscopy, which measures the fluorescence lifetime of a fluorophore to create an image. This lifetime is independent of intensity variations in the laser source and in background light changes. So its measurement improves image clarity.
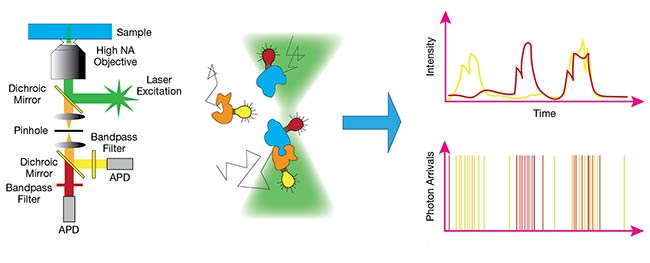
Single-photon detectors allow sensing and characterization of single molecules in fluorescence lifetime measurement (top). NA: numerical aperture.
Entangled photons are sent to a pair of quantum memories, where the photons are absorbed by the trapped atoms, thus transferring their entanglement to the memories (bottom). By monitoring the cavity for fluorescence from this cycling transition, users can reliably infer whether or not a single 795-nm photon has been absorbed by the atom in the cavity. Entangled photon states are at the heart of long-distance quantum teleportation studies. Photons represent the preferred option for long-distance entanglement distribution, while atomic or ionic quantum memories are potential storage and processing nodes for a quantum information network. Courtesy of Excelitas Technologies.
Time-correlated single-photon counting
is a common method for extracting fluorescent lifetime information while compensating for source and background variations. Systems using CMOS-based single-photon avalanche diode arrays allow wide-field imaging for fast analysis and potentially lower system cost.
In flow cytometry, fluorescence-activated cell sorting is used to separate particular cells or particles from a larger population. Single-photon detection allows the use of lower-power and therefore less-expensive lasers. It targets single-molecule or nanoparticle detection, where the amount of light emitted is extremely low.
The quantum world
Single photons also find use in many quantum technology applications. However, for these applications to become practical enough for the real world, reliable single-photon detectors will be needed.
Such detectors are in demand for banking, infrastructure, military, and other application sectors requiring secure communications systems that are impervious to hacking or intrusion. Quantum key distribution allows an information transmitter and receiver to create complex and highly secure cryptographic keys in real time, by employing the quantum properties of single photons. Once the key is created, users are free to encode information, knowing that communications are secure.
Quantum key distribution systems are moving from laboratories and dedicated link demonstrators to real-world deployments. As these systems transition to commercial applications, single-photon detectors will become critical to the systems’ success. While laboratory demonstrations relied on superconducting nanowires for detection, silicon and InGaAs APDs are the detectors of choice for deployed systems.
Quantum entanglement systems, another area of study in quantum technology, also rely on single-photon detection technology. Here too, APDs are becoming the detector of choice as systems move from the laboratory into commercial deployment.
The ability to detect and count single photons has enabled breakthroughs and discoveries in medical, industrial, aerospace, and military applications, among others. The action of photons in both chemical and physical reactions is useful for learning how energy and matter interact in all kinds of scenarios and under all kinds of conditions. Quantum technology applications are growing fast, and considerable potential exists for this game-changing technology to provide the information, imaging, and detection capabilities needed for future applications that have not yet even emerged.
Meet the author
Richard Simons is the product manager for low-light-level detection at Excelitas Technologies. He has an extensive background in photonics, with experience in high-power lasers, high-energy laser optics, fiber optics for telecommunications and sensors, lidar, and single-photon detection; email:
[email protected].