A prototype combining Raman spectroscopy and confocal autofluorescence imaging can identify basal cell carcinoma cells, providing key data for successful cancer treatment.
Radu Boitor, Hywel C. Williams and Ioan Notingher, University of Nottingham
A research team at the University of Nottingham has developed an autofluorescence-Raman spectroscopy technique that aims to streamline surgery to remove skin cancer. The technique has been trialed at the Nottingham University Hospitals NHS Trust. The scanning takes <40 min and provides a clear answer to whether the surgical margins are clear of basal cell carcinoma (BCC), which is a cancer that begins in the basal cell layer of the skin. The performance of the autofluorescence-Raman instrument was assessed in a recent clinical study on freshly excised skin specimens from 130 patients.
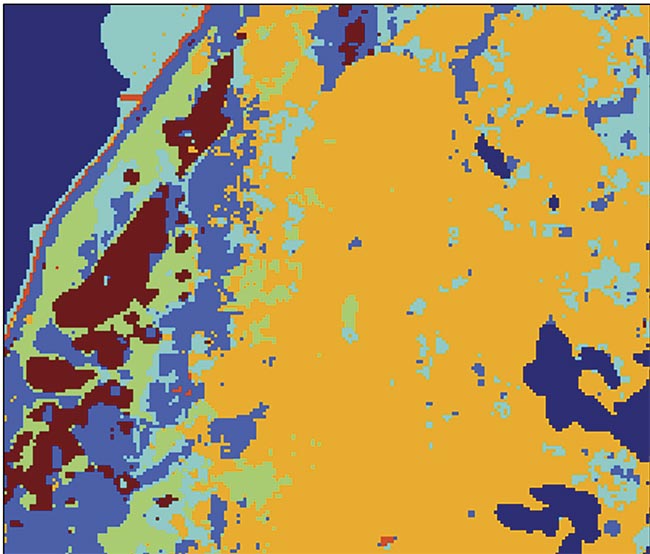
An image of a diagnostic test carried out with the aid of raster scanning Raman spectroscopy. The maroon color in the image represents basal cell carcinoma (BCC). Adapted with permission from Reference 3.
Current treatment
Human skin is composed of three main layers: the epidermis, the dermis, and the subcutaneous layer. The basal layer is a monolayer of cells that sits at the bottom of the epidermis. It continuously divides and produces the ever-changing cells that make up the epidermis. Prolonged and repeated exposure to ultraviolet radiation (such as from the sun) can induce mutations in these basal cells, causing them to proliferate excessively and invade the dermis. The resulting neoplasm is called BCC.
BCC is one of the most common skin cancers in the world, with approximately 6 million cases diagnosed each year: approximately 100,000 cases in the U.K., 300,000 in Australia, and 1.7 million in the U.S.1. The most common treatment for BCC is surgical removal via wide-local excision followed by a histopathological examination of the removed tissue. The treatment is deemed to be successful if the surgical margin is clear of cancerous cells after a microscopic histology examination. However, conventional histopathology takes around 1 to 2 weeks for wide-local excision surgery and only investigates 3% to 5% of the resection surface, resulting in many incomplete excisions of BCC. Such incomplete excisions have been linked to higher recurrence rates, which can be as high as 10% to 20% for high-risk BCC of the head and neck2.
For such high-risk BCCs, a more specialized surgical procedure called Mohs micrographic surgery can be used. In Mohs surgery, sequential thin layers of skin are removed and investigated by histopathology, until the entire tumor is cut out. The histological assessment is made via frozen sections, which can be completed within 60 min, allowing repeated surgical excisions for any residual tumor within the same day. This higher degree of certainty regarding the surgical margins ensures lower recurrence rates (1% to 2%) than for wide-local excision. However, processing and interpreting frozen section slides for Mohs surgery is resource-intensive and expensive, resulting in the technique not being widely available to all BCC patients. Therefore, a need has emerged for novel, less resource-intensive techniques that can detect residual BCC on excised tissue specimens in timescales comparable to or faster than frozen section histology, which could lead to wider adoption of margin-controlled surgery.
Raman and autofluorescence
The Raman effect occurs when an incident photon to a molecule has a higher energy than required for a vibrational transition, but a lower energy than required for an electronic transition. In such circumstances, one out of 1 million photons is inelastically scattered by the molecule, resulting in a photon that has an energy difference to the incident photons. This difference in energy corresponds to the vibrational energy modes of the molecule. Therefore, Raman spectroscopy can be used to determine the chemical structure of an investigated sample by investigating the position and intensity of the Raman spectral bands.
The biggest shortcoming of Raman spectroscopy when applied to medical applications is the relatively weak signal. Taking high-resolution measurements of tissue specimens measuring a few centimeters in size requires as long as several days. Such long scanning times are not compatible with the Mohs surgery workflow. To expedite measurement times, Raman spectroscopy can be paired with an additional technique to prescreen the tissue, such as reflectance confocal imaging, fluorescence imaging, or optical coherence tomography.
For skin specimens, autofluorescence confocal imaging with a 405-nm excitation laser was found by researchers to be an ideal complement to Raman spectroscopy. The main component of healthy dermis is collagen, which intrinsically fluoresces when illuminated with ~400-nm light3. The collection of fluorescent light >430 nm produces a map of collagen in the tissue surface, which highlights a large proportion of healthy tissue, allowing Raman spectroscopy to be used only in areas that do not contain collagen. A comparison of this approach with high-resolution Raman raster scans (10-µm resolution) showed that by directing Raman spectra acquisitions only to regions devoid of collagen, the number of required spectra to detect BCC is 100× less than for a raster scan (Figure 1).
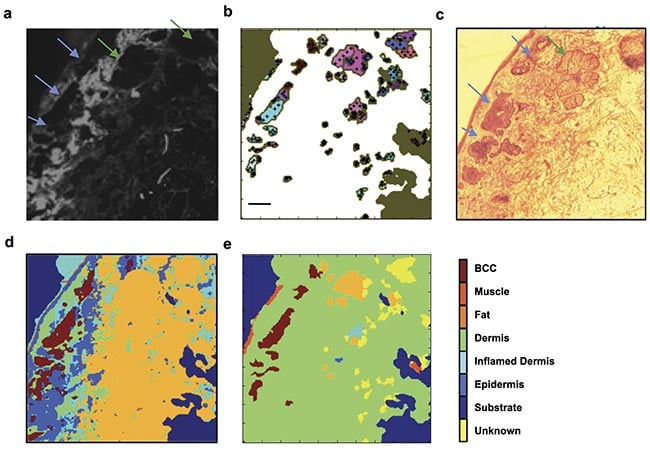
Figure 1. Selective sampling with Raman spectroscopy guided by autofluorescence confocal imaging. The tissue autofluorescence confocal image shows the distribution of collagen on the skin specimen (a); the automated image segmentation identifies regions with low autofluorescence signal and distributes sampling points in the region (black crosses, b); an H&E section showing the location of the tumor (blue arrows) and a sebaceous unit (green arrow, c); a diagnosis obtained by 200 × 200 raster-scanning Raman spectroscopy (40,000 spectra, d); a diagnosis obtained by combining autofluorescence imaging and Raman spectroscopy (350 spectra, e). The tumor is identified in the same location as in the higher-resolution raster scan and the H&E section. Scale bars: 0.5 mm. Adapted with permission from Reference 3.
For a centimeter-size specimen, this combined autofluorescence-Raman approach was able to investigate the entire resection margin of a typical Mohs skin specimen in <40 min, which was confirmed in the lab and by a larger-scale diagnostic test.
Combined assessment
These results led to the development of a fully automated prototype device in collaboration with RiverD International4. The instrument was developed so that it would be easy to use by medical personnel, require minimal sample processing and training, and be able to produce BCC detection maps showing BCC regions in a distinct color that do not require user interpretation (Figure 2). Typically, Raman spectroscopy systems are highly complex and require user training to extract results.
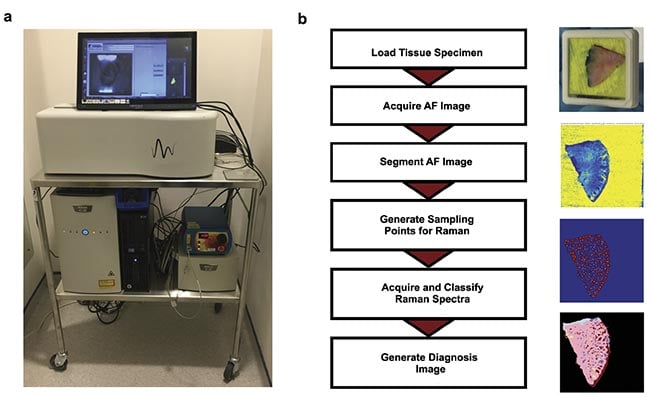
Figure 2. An autofluorescence-Raman instrument and measurement procedure. An autofluorescence-Raman instrument installed at the Nottingham University Hospitals NHS Treatment Centre (a). A flowchart of tissue processing and a measurement algorithm
(b). AF: autofluorescence. Courtesy of the University of Nottingham.
For measurements, Mohs layers were inserted into custom tissue cassettes, with the excision surface pressed against a transparent window (Figure 2b). Test autofluorescence-Raman measurements were carried out on frozen skin specimens that were formerly processed for Mohs surgery. These measurements showed that Raman spectroscopy can identify tumors with 100% sensitivity and a 92% specificity per spectrum. When translated to per tissue specimen level, the measurement maps showed tumors in the same locations as identified in the histology sections by the surgeons, which were blinded to the autofluorescence-Raman results.
Repeat measurements performed by users of varying experience produced comparable results on a set of seven specimens, confirming low inter-user variability (Figure 3).
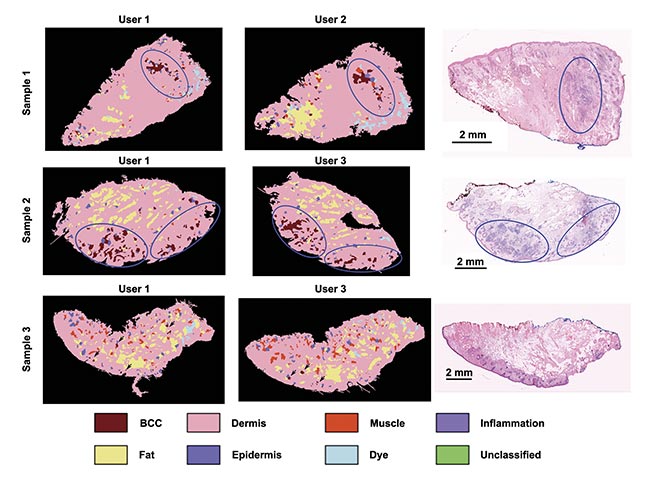
Figure 3. The consistency of an autofluorescence-Raman diagnosis among different users on frozen skin specimens. User 1: spectroscopy specialist; User 2: Mohs surgeon with 1-h instrument training; User 3: a core medical trainee with 8-h instrument training. Residual basal cell carcinoma (BCC) (blue ovals) and false-positive segments in Sample 3 (black arrows). Adapted with permission from Reference 4.
Mohs clinic
The promising results produced on frozen specimens led to the investigation of the autofluorescence-Raman device in the clinical pathway at the Nottingham University Hospitals NHS Treatment Centre, allowing intraoperative measurements of fresh skin specimens, immediately after excision and before processing for frozen section histology. The reference standard for assessing the performance of the instrument was a histological evaluation of the frozen sections by a panel of three dermatopathologists. To maintain the orientation of the tissue specimen with respect to the wound, colored-marker pen marks were made directly onto the cassette window (Figure 4a).
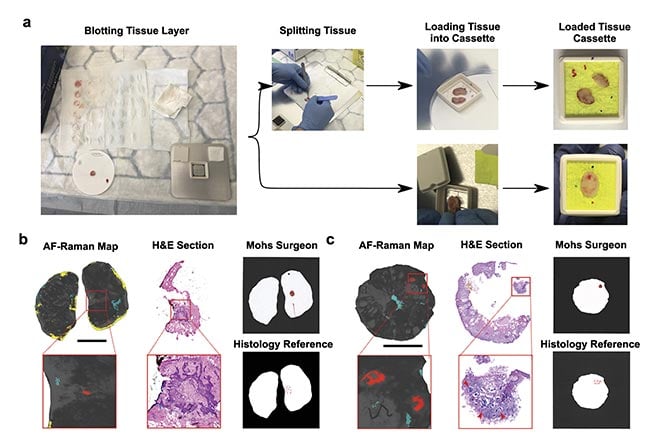
Figure 4. Tissue processing for autofluorescence-Raman measurements and representative split and “full-face” Mohs layers. Specimens are blotted to remove superficial blood and then placed directly in the cassette or split into several pieces and then placed in the cassette. Before closing the cassette, colored marks are made on the cassette window to preserve tissue orientation (a). The result for a “full-face” layer (b) and the result for a split layer (c). Red regions show basal cell carcinoma (BCC), blue regions show poor tissue-cassette window contact, and yellow regions indicate excessive blood. The BCC detections are confirmed by the hematoxylin and eosin (H&E) sections and the histology reference report. The Mohs surgeon report is also in concordance with the reference. Scalebar: 5 mm. AF: autofluorescence. Courtesy of
the University of Nottingham.
For the preliminary measurements in the clinic, Mohs layers were split into two or more pieces to relieve the tension in the specimens and improve flattening. This maximized the proportion of the excision surface in contact with the cassette window, and therefore the portion that is in focus for the autofluorescence-Raman measurements. In doing so, the technology was able to analyze an average of 96% of the resection surface for the 27 split layers investigated5. For this set of specimens, the instrument was able to detect a residual tumor with an 89% sensitivity (8/9 positive layers) and a 78% specificity (14/18 negative layers). For the same set of specimens, Mohs surgery produced an 89% sensitivity (8/9 positive layers) and an improved 94% specificity (17/18 negative layers).
Diagnostic test of accuracy
A formal diagnostic test of accuracy study was carried out on excised tissue specimens, or Mohs layers, from 130 patients with BCC. Mohs layers began to be processed exclusively as “full-face” at the recruitment center, rather than as split specimens (Figure 4). In doing so, the number of tissue samples that needed to be sectioned by histology was reduced.
From the 130 measurements performed, 125 were successful and 5 produced an error: There were three cases of incorrect placements of the cassette and two cases of the incorrect software version being loaded. All errors were caused by the instrument user, rather than a fault with the instrument.
For each incomplete measurement, the instrument returned an error, rather than an incorrect measurement result.
The first metric that was calculated from the autofluorescence-Raman image was the proportion of the full-face resection surface that was investigated by the instrument. The results showed that, on average, the instrument scanned 91% of the surgical margin, a decrease of 5% in the investigated surface area over the split specimens6. As expected, this had a significant effect on the sensitivity of the detection of BCC. The technique was able to detect a residual tumor with a 67% sensitivity (24/36 positive layers) and a 73% specificity (65/89 negative layers) according to the reference standard of consensus histopathology. Mohs surgery produced an 86% sensitivity (31/36 positive layers) and an 89% specificity (79/89 negative layers).
Upon further investigation, 11 out of the 12 false-negative cases were caused either by the presence of blood or inadequate tissue-cassette window contact. If cases for which no Raman signal could be captured from the tumor on the excision surface due to obstructions are omitted, autofluorescence-Raman-detection sensitivity increases to 96%. This is comparable to the 89% sensitivity observed for split layers — a method that achieved 96% surface area coverage.
Future prospects
The preliminary study and the diagnostic test of accuracy showed that the autofluorescence-Raman instrument can detect residual BCC with ~90% sensitivity, provided that the specimen is in good contact with the cassette coverslip and is clear of residual blood. In addition to replacing frozen section histopathology in Mohs centers, when tissue contact problems have been overcome, the instrument could also be used as a method of margin control during wide-local excision of BCC in centers without access to Mohs surgery, including low-resource areas as well as large portions of Europe.
As the technology provides an objective diagnosis, it could be used by any member of the surgery team directly on the fresh specimens, without the need of complex histology facilities and without the delays that patients and doctors experience while obtaining results. Future work will be focused on adapting the tissue processing procedure to reduce the amount of superficial blood and to better flatten the tissue. Further development will also incorporate the ability to detect other skin cancers, such as squamous cell carcinoma, which would further increase its applicability in the clinic.
Meet the authors
Radu Alex Boitor is a research associate in the Biophotonics Lab at the University of Nottingham in the U.K. He received his bachelor’s and master’s degrees in medical physics from Babes-Bolyai University in Cluj-Napoca, Romania. He received his Ph.D. from the University of Nottingham, working on the development and optimization of the autofluorescence-Raman spectroscopy device; email: [email protected].
Hywel C. Williams trained in dermatology and epidemiology in London. There, he developed diagnostic criteria for eczema that are now the most widely used in the world. Williams joined the University of Nottingham in 1994 where he also worked as a consulting pediatric dermatologist until 2023. He now codirects the Centre of Evidence-Based Dermatology with Kim Thomas; email: [email protected].
Ioan Notingher graduated from Babes-Bolyai University in Cluj-Napoca, Romania, and received his Ph.D. at London South Bank University (Photophysics Research Group). This was followed by postdoctoral research at Imperial College London and Edinburgh University. He was appointed lecturer in 2006 at the University of Nottingham, where he established the Biophotonics Group; email: [email protected].
References
1. C. Fitzmaurice et al. (2019). Global Burden of Disease Cancer Collaboration. Global, Regional, and National Cancer Incidence, Mortality, Years of Life Lost, Years Lived With Disability, and Disability-Adjusted Life-Years for
29 Cancer Groups, 1990 to 2017: A Systematic Analysis for the Global Burden of Disease Study. JAMA Oncol, Vol. 5,
No. 12, pp. 1749-1768.
2. A. Kimyai-Asadi et al. (2005). Efficacy of narrow-margin excision of well-demarcated primary facial basal cell carcinomas. J Am Acad Dermatol, Vol. 53, No. 3, pp. 464-468.
3. K. Kong et al. (2013). Diagnosis of tumors during tissue-conserving surgery with integrated autofluorescence and Raman scattering microscopy. Proc Natl Acad Sci USA, Vol. 110, No. 38, pp. 15189-15194.
4. R. Boitor et al. (2017). Automated multimodal spectral histopathology for quantitative diagnosis of residual tumour during basal cell carcinoma surgery. Biomed Opt Express, Vol. 8,
No. 12, pp. 5749-5766.
5. R. Boitor et al. (2024). Ex vivo assessment of basal cell carcinoma surgical margins in Mohs surgery by autofluorescence-Raman spectroscopy: A pilot study. JEADV Clin Pract, Vol. 3, No. 2,
pp. 498-507.
6. R. Boitor et al. (2024). Diagnostic accuracy of autofluorescence-Raman microspectroscopy for surgical margin assessment during Mohs micrographic surgery of basal cell carcinoma, Br J
Dermatol, Vol. 191, No. 3, pp. 428-436.